
An official website of the United States government
The .gov means it’s official. Federal government websites often end in .gov or .mil. Before sharing sensitive information, make sure you’re on a federal government site.
The site is secure. The https:// ensures that you are connecting to the official website and that any information you provide is encrypted and transmitted securely.
- Publications
- Account settings
- My Bibliography
- Collections
- Citation manager

Save citation to file
Email citation, add to collections.
- Create a new collection
- Add to an existing collection
Add to My Bibliography
Your saved search, create a file for external citation management software, your rss feed.
- Search in PubMed
- Search in NLM Catalog
- Add to Search
The continued need for animals to advance brain research
Affiliations.
- 1 Radboud University Medical Center, Nijmegen, the Netherlands. Electronic address: [email protected].
- 2 University Medical Center Utrecht, Utrecht, the Netherlands.
- 3 The Max Delbrück Center for Molecular Medicine in the Helmholtz Association (MDC), Berlin, Germany.
- 4 Simons Initiative for the Developing Brain, University of Edinburgh, Edinburgh EH8 9XD, UK; Center for Translational Neuromedicine, University of Copenhagen, 2200 Copenhagen, Denmark.
- 5 KU Leuven, Leuven Brain Institute and Faculty of Psychology and Educational Sciences, Leuven, Belgium.
- 6 School of Psychology, UNSW Sydney, Sydney, NSW, Australia.
- 7 Maastricht University, Maastricht, the Netherlands.
- 8 Federal University of Santa Maria, Santa Maria, Brazil.
- 9 Groningen Institute for Evolutionary Life Sciences, University of Groningen, Groningen, the Netherlands.
- 10 Erasmus Medical Center, Rotterdam, the Netherlands.
- 11 Radboud University, Nijmegen, the Netherlands.
- 12 Cornell University, Ithaca, NY, USA.
- 13 Swammerdam Institute for Life Sciences, Faculty of Science, University of Amsterdam, Amsterdam, the Netherlands.
- 14 Amsterdam University Medical Center, University of Amsterdam, Amsterdam, the Netherlands; Amsterdam University Medical Center, Free University, Amsterdam, the Netherlands.
- 15 Central Institute of Mental Health, University of Heidelberg, Mannheim Faculty, Mannheim, Germany.
- 16 Radboud University Medical Center, Nijmegen, the Netherlands.
- 17 Institute of Veterinary Pathology, Gießen, Gießen, Germany; Center of Mind Brain and Behavior (CMBB), Philipps-University of Marburg and Justus-Liebig-University Gießen, Marburg, Germany.
- 18 Understanding Animal Research, London, UK.
- 19 Institute of Behavioural Neuroscience, University College London, London WC1H 0AP, UK.
- 20 Institute of Experimental Endocrinology, Biomedical Research Center, Slovak Academy of Sciences, Bratislava, Slovakia.
- 21 Netherlands Institute for Neuroscience (NIN), Amsterdam, the Netherlands; Amsterdam University Medical Center, University of Amsterdam, Amsterdam, the Netherlands.
- 22 Faculty of Science, Utrecht University, Utrecht, the Netherlands.
- 23 Mayo Clinic, Rochester, MN, USA.
- 24 European Animal Research Association, London, UK.
- 25 Division of Molecular Psychiatry, Center of Mental Health, University of Würzburg, Würzburg, Germany; Laboratory of Psychiatric Neurobiology, Institute of Molecular Medicine, Sechenov First Moscow State Medical University, Moscow, Russia; Department of Neuropsychology and Psychiatry, School for Mental Health and Neuroscience (MHeNS), Maastricht University, Maastricht, the Netherlands.
- 26 Faculty of Veterinary Medicine, Utrecht University, Utrecht, the Netherlands.
- 27 Faculty of Biology and Medicine, University of Lausanne, Lausanne, Switzerland.
- 28 University of Oxford, Oxford, UK.
- 29 University of Pisa, Pisa, Italy.
- 30 Maj Institute of Pharmacology, Polish Academy of Sciences, Kraków 31-343, Poland.
- 31 Instituto Cajal, Consejo Superior de Investigaciones Científicas, Madrid, Spain.
- 32 Brain Institute, Federal University of Rio Grande do Norte, Natal, Brazil.
- 33 Department of Physiology, Federal University of Rio Grande do Norte, Natal, Brazil.
- 34 Salari Institute of Cognitive and Behavioral Disorders (SICBD), Karaj, Alborz, Iran.
- 35 Center for Neurogenomics and Cognitive Research, Vrije Universiteit Amsterdam, Amsterdam, the Netherlands.
- 36 Danish Research Institute of Translational Neuroscience - DANDRITE, Nordic-EMBL Partnership for Molecular Medicine, Aarhus University, Aarhus C, Denmark.
- 37 Sleep and Circadian Neuroscience Institute, University of Oxford, Oxford, UK.
- 38 Biology Department, Faculty of Science, Utrecht University, Utrecht, the Netherlands.
- 39 Center of Mind Brain and Behavior (CMBB), Philipps-University of Marburg and Justus-Liebig-University Gießen, Marburg, Germany; Philipps-University of Marburg, Faculty of Psychology, Experimental and Biological Psychology, Behavioral Neuroscience, Marburg, Germany; KU Leuven, Leuven Brain Institute and Faculty of Psychology and Educational Sciences, Leuven, Belgium.
- 40 Radboud University, Nijmegen, the Netherlands. Electronic address: [email protected].
- PMID: 34352213
- DOI: 10.1016/j.neuron.2021.07.015
Policymakers aim to move toward animal-free alternatives for scientific research and have introduced very strict regulations for animal research. We argue that, for neuroscience research, until viable and translational alternatives become available and the value of these alternatives has been proven, the use of animals should not be compromised.
Copyright © 2021 Elsevier Inc. All rights reserved.
PubMed Disclaimer
Similar articles
- Animal Research in Neuroscience: A Duty to Engage. Bennett AJ, Ringach DL. Bennett AJ, et al. Neuron. 2016 Nov 2;92(3):653-657. doi: 10.1016/j.neuron.2016.10.034. Neuron. 2016. PMID: 27810011
- Ethical issues associated with the use of animal experimentation in behavioral neuroscience research. Ohl F, Meijboom F. Ohl F, et al. Curr Top Behav Neurosci. 2015;19:3-15. doi: 10.1007/7854_2014_328. Curr Top Behav Neurosci. 2015. PMID: 25023419 Review.
- The role of MRI in applying the 3Rs to non-human primate neuroscience. Prescott MJ, Poirier C. Prescott MJ, et al. Neuroimage. 2021 Jan 15;225:117521. doi: 10.1016/j.neuroimage.2020.117521. Epub 2020 Nov 1. Neuroimage. 2021. PMID: 33137476
- Does the goal justify the methods? Harm and benefit in neuroscience research using animals. Vieira de Castro AC, Olsson IA. Vieira de Castro AC, et al. Curr Top Behav Neurosci. 2015;19:47-78. doi: 10.1007/7854_2014_319. Curr Top Behav Neurosci. 2015. PMID: 24844681 Review.
- Neuroethics and Animals: Report and Recommendations From the University of Pennsylvania Animal Research Neuroethics Workshop. Shriver AJ, John TM. Shriver AJ, et al. ILAR J. 2021 Sep 24;60(3):424-433. doi: 10.1093/ilar/ilab024. ILAR J. 2021. PMID: 34370840 Free PMC article.
- Cross-species analysis uncovers the mitochondrial stress response in the hippocampus as a shared mechanism in mouse early life stress and human depression. Hofstra BM, Hoeksema EE, Kas MJ, Verbeek DS. Hofstra BM, et al. Neurobiol Stress. 2024 May 14;31:100643. doi: 10.1016/j.ynstr.2024.100643. eCollection 2024 Jul. Neurobiol Stress. 2024. PMID: 38800537 Free PMC article.
- Towards improved screening of toxins for Parkinson's risk. Shan L, Heusinkveld HJ, Paul KC, Hughes S, Darweesh SKL, Bloem BR, Homberg JR. Shan L, et al. NPJ Parkinsons Dis. 2023 Dec 19;9(1):169. doi: 10.1038/s41531-023-00615-9. NPJ Parkinsons Dis. 2023. PMID: 38114496 Free PMC article. Review.
- Wiring and Volume Transmission: An Overview of the Dual Modality for Serotonin Neurotransmission. Gianni G, Pasqualetti M. Gianni G, et al. ACS Chem Neurosci. 2023 Dec 6;14(23):4093-4104. doi: 10.1021/acschemneuro.3c00648. Epub 2023 Nov 15. ACS Chem Neurosci. 2023. PMID: 37966717 Free PMC article. Review.
- Evidence-based severity assessment of the forced swim test in the rat. Becker L, Mallien AS, Pfeiffer N, Brandwein C, Talbot SR, Bleich A, Palme R, Potschka H, Gass P. Becker L, et al. PLoS One. 2023 Oct 12;18(10):e0292816. doi: 10.1371/journal.pone.0292816. eCollection 2023. PLoS One. 2023. PMID: 37824495 Free PMC article.
- A comparative review on neuroethical issues in neuroscientific and neuroethical journals. Ishida S, Nishitsutsumi Y, Kashioka H, Taguchi T, Shineha R. Ishida S, et al. Front Neurosci. 2023 Sep 14;17:1160611. doi: 10.3389/fnins.2023.1160611. eCollection 2023. Front Neurosci. 2023. PMID: 37781239 Free PMC article. Review.
- Search in MeSH
Grants and funding
- 110157/Z/15/Z/WT_/Wellcome Trust/United Kingdom
LinkOut - more resources
Full text sources.
- Elsevier Science
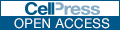
- Citation Manager
NCBI Literature Resources
MeSH PMC Bookshelf Disclaimer
The PubMed wordmark and PubMed logo are registered trademarks of the U.S. Department of Health and Human Services (HHS). Unauthorized use of these marks is strictly prohibited.
Search form
What makes the human brain different yale study reveals clues.

(© stock.adobe.com)
What makes the human brain distinct from that of all other animals — including even our closest primate relatives? In an analysis of cell types in the prefrontal cortex of four primate species, Yale researchers identified species-specific — particularly human-specific — features, they report Aug. 25 in the journal Science.
And they found that what makes us human may also makes us susceptible to neuropsychiatric diseases.
For the study, the researchers looked specifically at the dorsolateral prefrontal cortex (dlPFC), a brain region that is unique to primates and essential for higher-order cognition. Using a single cell RNA-sequencing technique, they profiled expression levels of genes in hundreds of thousands of cells collected from the dlPFC of adult humans, chimpanzees, macaque, and marmoset monkeys.
“ Today, we view the dorsolateral prefrontal cortex as the core component of human identity, but still we don’t know what makes this unique in humans and distinguishes us from other primate species.” said Nenad Sestan, the Harvey and Kate Cushing Professor of Neuroscience at Yale, professor of comparative medicine, of genetics. and of psychiatry, and the lead senior author of the paper. “Now we have more clues.”
To better understand these differences, the researchers first asked whether there are there any cell types uniquely present in humans or other analyzed non-human primate species. After grouping cells with similar expression profiles they revealed 109 shared primate cell types but also five that were not common to all species. These included a type of microglia, or brain-specific immune cell, that was present only in humans and a second type shared by only humans and chimpanzees.
The human-specific microglia type exists throughout development and adulthood, the researchers found, suggesting the cells play a role in maintenance of the brain upkeep rather than combatting disease.
“ We humans live in a very different environment with a unique lifestyle compared to other primate species; and glia cells, including microglia, are very sensitive to these differences,” Sestan said. “The type of microglia found in the human brain might represent an immune response to the environment.”
An analysis of gene expression in the microglia revealed another human-specific surprise — the presence of the gene FOXP2. This discovery raised great interest because variants of FOXP2 have been linked to verbal dyspraxia, a condition in which patients have difficulty producing language or speech. Other studies have also shown that FOXP2 is associated with other neuropsychiatric diseases, such as autism, schizophrenia, and epilepsy.
Sestan and colleagues found that this gene exhibits primate-specific expression in a subset of excitatory neurons and human-specific expression in microglia.
“ FOXP2 has intrigued many scientists for decades, but still we had no idea of what makes it unique in humans versus other primate species,” said Shaojie Ma, a postdoctoral associate in Sestan’s lab and co-lead author. We are extremely excited about the FOXP2 findings because they open new directions in the study of language and diseases.”
The research was funded by the National Institutes of Health and National Institute of Mental Health.
Other authors include co-lead author Mario Skarica, associate research scientist in neuroscience at Yale School of Medicine; co-senior author Andre Sousa, assistant professor of neuroscience at the University of Wisconsin-Madison; and co-senior author Stephen M. Strittmatter, the Vincent Coates Professor of Neurology and professor of neuroscience at Yale, chair of the Department of Neuroscience, and director of the Kavli Institute for Neuroscience.
Health & Medicine
Media Contact
Bess Connolly : [email protected] ,

Yale carbon capture center takes next steps in leadership, research

1stGenYale alumni share perspectives on navigating Yale and beyond

Olympic lessons: leadership and fun

The Cowles 2024 Summer Conferences welcome over 350 economists to Yale
- Show More Articles
December 1, 2008
13 min read
One World, Many Minds: Intelligence in the Animal Kingdom
We are used to thinking of humans as occupying the sole pinnacle of evolutionary intelligence. That's where we're wrong
By Paul Patton
We were talking about politics. My housemate, an English professor, opined that certain politicians were thinking with their reptilian brains when they threatened military action against Iran. Many people believe that a component of the human brain inherited from reptilian ancestors is responsible for our species’ aggression, ritual behaviors and territoriality.
One of the most common misconceptions about brain evolution is that it represents a linear process culminating in the amazing cognitive powers of humans, with the brains of other modern species representing previous stages. Such ideas have even influenced the thinking of neuroscientists and psychologists who compare the brains of different species used in biomedical research. Over the past 30 years, however, research in comparative neuroanatomy clearly has shown that complex brains—and sophisticated cognition—have evolved from simpler brains multiple times independently in separate lineages, or evolutionarily related groups: in mollusks such as octopuses, squid and cuttlefish; in bony fishes such as goldfish and, separately again, in cartilaginous fishes such as sharks and manta rays; and in reptiles and birds. Nonmammals have demonstrated advanced abilities such as learning by copying the behavior of others, finding their way in complicated spatial environments, manufacturing and using tools, and even conducting mental time travel (remembering specific past episodes or anticipating unique future events). Collectively, these findings are helping scientists to understand how intelligence can arise—and to appreciate the many forms it can take.
The Tree of Life To understand why a new view of the evolution of brains and minds is only now coming to full fruition, it is useful to review historical notions. Medieval naturalists placed living things along a linear scale called the great chain of beings, or scala naturae . This hierarchical sequence ranked creatures such as worms and slugs as lowly and humans as the highest of earthly beings. In the late 1800s the enormous mass of evidence contained in Charles Darwin’s masterwork, On the Origin of Species , convinced most of his scientific contemporaries that evolution was a reality. Darwin explained that modern species were related by physical descent and saw the relations among species as resembling the diverging branches of a family genealogical tree. Few, however, fully grasped the revolutionary implications of this tree of life—in which modern species represent the tips of the branches and inner branches represent past species, forming junctions where two lineages branch from a common ancestor.
On supporting science journalism
If you're enjoying this article, consider supporting our award-winning journalism by subscribing . By purchasing a subscription you are helping to ensure the future of impactful stories about the discoveries and ideas shaping our world today.
So when comparative neuroanatomy first blossomed at the end of the 19th century, most researchers interpreted its findings in terms of the old linear scale. They believed modern invertebrates (animals without backbones), fish, amphibians, reptiles, birds, mammals and humans to be living representatives of successive evolutionary steps toward a more complex brain, with new brain components added at each step. Given the relative lack of interest in comparative neuroanatomy during the mid-20th century, these ideas persisted unchallenged for decades. The traditional ideas about sequential brain evolution appeared, for example, in the late neuroscientist and psychiatrist Paul D. MacLean’s triune brain model, formulated in the 1960s. MacLean’s model promoted the belief that the human brain contains a “reptilian complex” inherited from reptilian ancestors.
Beginning in the 1980s, the field of comparative neuroanatomy experienced a renaissance. In the intervening decades evolutionary biologists had learned a great deal about vertebrate evolutionary history, and they developed new and effective methods of applying Darwin’s concept of the tree of life to analyze and interpret their findings. It is now apparent that a simple linear hierarchy cannot adequately account for the evolution of brains or of intelligence. The oldest known multicellular animal fossils are about 700 million years old. By the Cambrian period, about 520 million years ago, the animal kingdom had branched into about 35 major groups, or phyla, each with its own distinctive body plan. As a separate branch of the tree of life, each lineage continued to evolve and diversify independently of the others. Complex brains evolved independently in multiple phyla, notably among the cephalopod mollusks of the phylum Mollusca and, of course, among various groups of vertebrates. Vertebrate evolution has likewise involved repeated branching, with complex brains evolving from simpler brains independently along numerous branches.
Alien Minds Cephalopod mollusks, a group that includes octopuses, squid and cuttlefish, have evolved the most sophisticated nervous system of all invertebrates—and their cognitive abilities reflect that complexity. The brain of an octopus contains an estimated 170 million neurons, a number comparable to that of the brains of some vertebrates. In relation to body size, this brain is as large as that of some birds. Having evolved independently in another phylum, the structure of the octopus’s brain looks utterly alien as compared with the more familiar brains of vertebrates. The exquisitely sensitive and flexible tentacles of the octopus contain as many neurons as its brain does, and severed tentacles remain capable of coordinated movements.
Behavioral studies show that octopuses can distinguish and classify objects based on size and shape, much as rats do. They can learn to navigate simple mazes and to solve problems, such as removing a tasty food item from a sealed container. In 1992 two Italian neuroscientists, Graziano Fiorito of the Dohrn Zoological Station in Naples and Pietro Scotto, then at the University of Reggio Calabria in Catanzaro, published surprising evidence that an octopus can learn to accomplish a task by watching another octopus perform it. They trained octopuses to choose between a red ball and a white ball. If the octopus opted for the correct ball, it got a piece of fish as reward. If it selected incorrectly, it received a mild electric shock as punishment.
Once the training was completed, the investigators let an untrained octopus watch a trained animal perform the task from behind a glass barrier. The untrained animals did monitor the trained animals, as indicated by movements of their head and eyes. When allowed to select between the two balls themselves, the observer octopuses then made correct choices, which they could only have learned by watching. The ability to learn by studying others has been regarded as closely related to conceptual thought.
Undersea Smarts Unlike the octopus, bony fishes and their cartilaginous cousins are fellow vertebrates and seafaring members of our own phylum, Chordata. Research in the past few years has shown that these animals display some cognitive abilities once thought unique to mammals. In a series of studies starting in 1994, a team of investigators at the University of Seville in Spain tested the spatial smarts of goldfish, a familiar bony fish. The goldfish swam through watery versions of mazes such as those traditionally used to test similar cognitive skills in rats. They showed many of the same basic spatial abilities that rodents do, including the ability to use distant visual cues to find a particular place, even when the surrounding maze has been reoriented.
The forebrains of fishes endow them with these abilities. The forebrains of most vertebrates also directly receive and process smell information. Early comparative neuroanatomists, guided by their belief in a linear evolutionary scale, thought the forebrains of “primitive” fishes and amphibians were olfactory centers that did little else. We now know that, as in mammals, the forebrains of fishes and amphibians receive the full panoply of sensory information. The main modern group of bony fishes, the teleosts, first appeared about 200 million years ago, well after vertebrates ancestral to humans had emerged onto land, further proof of the independent development of their intelligence. In body-relative terms, the brains of these fishes are often comparable in size to those of land-dwelling reptiles. In the old phylogenetic scale, fish were considered “lower” than reptiles.
Cartilaginous fishes constitute a separate lineage from bony fishes, and their defining trait is a skeleton consisting of cartilage. Modern examples of this group include sharks, skates and rays. Though once regarded as primitive, some members of this lineage have evolved the largest brains in relation to their bodies of any nonmammalian aquatic vertebrate. In 2005 neuroethologists Vera Schluessel and Horst Bleckmann, both at the University of Bonn in Germany, repeated some of the Spanish group’s spatial tests on the freshwater stingray. It exhibited place-finding abilities akin to those found in goldfish.
By performing tests on goldfish after parts of their forebrain had been destroyed, the Spanish team showed in a study published in 2006 that the spatial abilities of goldfish derive from a part of the roof, or pallium, of the forebrain that may correspond to the hippocampus in mammals. Together these new studies indicate that the common ancestor of cartilaginous fishes, bony fishes and land vertebrates may already have possessed a hippocampuslike structure and the spatial cognitive abilities it confers. The hippocampus, which is also involved in processing emotions, is the main pallial component of the limbic system; in MacLean’s triune brain scheme, it was supposed to have originated with mammals. A variety of other limbic system structures are now known to exist in nonmammals.
Birds and Reptiles When a lineage of bony fishes left the seas for land about 365 million years ago, it eventually gave rise to all the four-limbed land vertebrates alive today—and two major types of brain organizational plans. These vertebrates branched into two main groups. The first group, the synapsids, appeared 320 million years ago and eventually evolved into modern mammals, whereas the second, the sauropsids, appeared 10 million years later and evolved into modern birds and reptiles (as well as the extinct dinosaurs). In their 300 million years of separate brain evolution, some members of each of the two groups have evolved quite sophisticated cognitive abilities based on very different forebrain organizational plans.
This difference in forebrain organization initially caused confusion among comparative neuroanatomists. When seen in cross section, each hemisphere of the vertebrate forebrain consists of a mass of neural tissue surrounding a central fluid-filled cavity called the ventricle. The forebrains of reptiles and birds include a prominent mass of neural tissue that bulges into this ventricle, in some cases largely obliterating it. Early comparative neuroanatomists mistook this bulge for a part of the basal ganglia, a structure in the floor of the forebrain. They concluded that the forebrains of reptiles and birds were dominated by the basal ganglia and possessed only a rudimentary pallium. The pallium is the structure that has elaborated into the cerebral cortex in mammals. Pioneering behavioral studies reinforced the interpretation suggested by the apparently rudimentary pallium. “The bird, its brain dominated by its basal nuclei, is essentially a highly complex mechanism with little learning capacity,” concluded comparative anatomist Alfred Romer in 1955. As it turns out, these seemingly consistent neuroanatomical and behavioral findings were both mistaken.
A series of comparative neuroanatomical studies in the 1960s, beginning with the work of Harvey J. Karten, now at the University of California, San Diego, has conclusively shown that the bulging mass of neural tissue in sauropsid forebrains, now known as the dorsal ventricular ridge (DVR), is not a part of the basal ganglia. It is instead a part of the pallium and appears to be the sauropsid counterpart of the mammalian neocortex. In mammals the neocortex is the largest part of the pallium and is involved in sophisticated cognitive abilities such as executive planning, learning and memory, reasoning, fine-motor control and perception; in humans it accounts for language. The basal ganglia in fact make up no larger part of the forebrain in sauropsids than they do in mammals. Mammals have nothing like the DVR. Neuroanatomical terminology for birds was revised to reflect this new awareness only in 2002.
The neocortex of mammals and the DVR of reptiles and birds are dramatically different in structure. The former is an extended thin sheet of tissue, with nerve cells organized into layers and with different territories of the sheet essentially performing different functions. The latter is a bulk mass of neural tissue structured into a series of clusters of nerve cells, or nuclei, with nuclei specialized for various functions. Despite these structural differences, the neocortex and DVR share similar connections to other parts of the brain as well as apparently similar cognitive functions. For example, there is now evidence that a part of the DVR in birds, called the nidopallium caudolaterale, may be involved in planning and executive control of behavior, much like the frontal lobes of the neocortex in mammals. In its internal structure and connections with other brain parts, the DVR in reptiles is generally simpler than that in birds. Despite their common forebrain plan, birds typically have much larger forebrains in relation to their bodies than reptiles do.
Far from being “birdbrains,” our feathered friends have displayed clever behaviors. Among birds, the largest forebrains are those of parrots and corvids (a group that includes crows, jays, ravens and jackdaws). Relative to body size, the brain of a parrot is as large as that of a chimpanzee, although, in absolute terms, it is about the size of a walnut. In recent years researchers have documented stunning cognitive abilities in these two groups of birds.
In the wild, for example, New Caledonian crows manufacture two types of simple tools to gain access to otherwise unobtainable foods. They trim and sculpt twigs to fashion hook tools to poke out insect larvae from holes in trees. And they make probes for finding insects under leaf detritus by stripping off pieces of the barbed pandanus leaves to sharpen them to a point. Psychologists Gavin Hunt and Russell Gray, both at the University of Auckland in New Zealand, reported in 2003 that New Caledonian crows’ tools have some features that appear more sophisticated than those of chimpanzees. The crows can craft a diverse variety of tools, modified by innovation from a common design. They can add cumulative improvements to their tools and can teach other members of their group to copy good designs faithfully.
Nicola S. Clayton, now at the University of Cambridge, has demonstrated, in a series of papers beginning in 1998, striking cognitive abilities in the Florida scrub jay, another type of corvid. These birds stash food in hundreds of different hidden caches dispersed over a wide area. They can remember the locations of all their hoards and retrieve food from them at a later time. Nonperishable foods, such as seeds, may remain in storage for months on end. Perishable foods, such as grubs and worms, must be retrieved just hours or days later.
Clayton and her students were able to use this naturally occurring behavior to show that Florida scrub jays can recall specific episodes in their past. The birds were provided with perishable worms and nonperishable nuts, which they cached in the individual compartments of sand-filled ice cube trays. They cached in different trays on different days and were then denied access to the trays for a specified period. If the birds could not access the trays for a short time, they should have tried to retrieve the worms, which are their preferred food, from the appropriate compartments of the appropriate trays. On the other hand, if the birds were denied access to the trays for a longer time, the worms no longer would have been fresh, and the jays should have tried to retrieve the nuts. To solve this problem, the birds needed to recall what they cached, where they cached it and when they did so. The birds successfully performed this complex task. Such an ability has yet to be demonstrated in a nonhuman mammal.
Even more amazingly, Clayton showed that the birds can anticipate unique future events. She allowed jays to observe others of their kind cache food and then permitted them to pilfer the caches. Later these birds cached their own food, either alone or in the presence of another jay. Birds that had acted as thieves took great precautions to conceal their food-caching activities when in the presence of another jay. Although the jays had experienced food theft only in the role of thief, they nonetheless were able to imagine themselves in the role of victim. The ability to recall specific episodes in the past and to predict future occurrences is known as mental time travel [see “ Intelligence Evolved ,” by Ursula Dicke and Gerhard Roth; Scientific American Mind , August/September 2008]. Before Clayton’s work, this cognitive ability was thought to be unique to humans.
Perhaps most stunning, an African gray parrot named Alex became famous for his ability to name 50 different objects. Alex learned the labels for seven colors and five shapes. In 1996 psychologist Irene M. Pepperberg, then at the University of Arizona, reported that Alex could classify objects by color and shape. Alex could ask for objects by name using phrases such as “want banana.” Alex even learned number labels from one to six and seemed to grasp the concept of zero, as evidenced by an appropriate use of “none.” A host of control experiments showed that Alex’s feats were genuinely cognitive and not the result of simple conditioned learning. Similar cognitive abilities had never been demonstrated outside humans and their closest primate relatives [see “ Bird Brains? Hardly ,” by Christine Scholtyssek; Scientific American Mind , April/May 2006].
Although scientists have yet to discover birdlike cognitive abilities in reptiles, the view of them as instinct-driven automatons appears likewise to have been misconceived. Reptiles are the victims of biased intelligence tests. Mammals, with their high and constant body temperatures, must incessantly seek food to fuel their energy-costly metabolism. They can thus easily be induced to perform all manner of learning tasks for a food reward. Reptiles lack a comparably powerful demand for food and often perform poorly when it is offered as a reward. They are now known to exhibit a variety of forms of simple learning when provided with species-appropriate rewards, such as the warmth of a sun lamp. Experiments with spatial mazes, for example, have demonstrated that turtles possess spatial skills similar to those described for fishes earlier, including the ability to find a particular place based on distant visual cues, despite rotational displacement of a maze.
Scientists still do not have answers for a great many questions about animal intelligence and its evolution. A major problem involves the identification of species-appropriate tests of cognition. Clayton’s demonstration of mental time travel in Florida scrub jays exploited a naturally occurring behavior of that species. We will not know whether this knack is an unusual quirk of scrub jays and other food-caching birds or a widespread capacity until behaviorally appropriate tasks are identified for other species. The cognitive facilities demonstrated for birds, mammals and cephalopod mollusks depend on very different nervous systems. What allows them all to serve similar cognitive functions? Our understanding of intelligence and the brain in nonmammals is still in its infancy.
In recent decades scientists have cast aside a linear, sequential view of brain evolution in which the human brain incorporates components resembling the brains of modern fishes, amphibians, reptiles and birds and have adopted a new view of divergently branching brain and mind evolution. Substantial cognitive abilities have evolved multiple times, based on differing neural substrates—including the mental agility that enables us humans to decipher brain evolution and its meaning.
Note: This article was originally printed with the title, "One World, Many Minds".
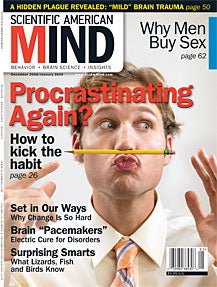

What animal minds can tell us about our own
The Cognitive Evolution Group at the University of Michigan takes a comparative approach to primate research to understand where complex cognition comes from
Vol. 51, No. 1 Print version: page 94
- Cognition and the Brain
- Animal Research
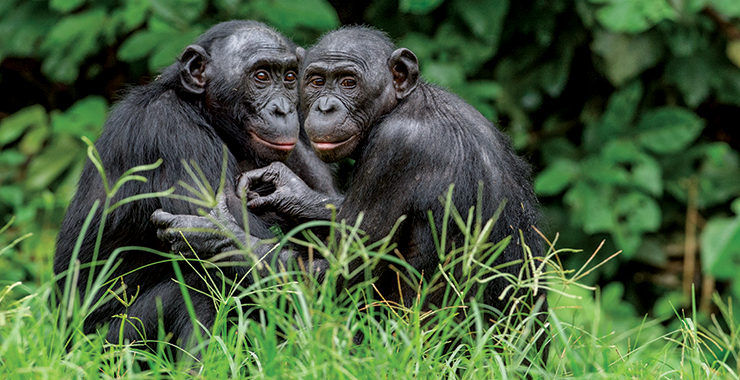
Can a chimpanzee cook you dinner? It sounds like a sitcom setup, but Alexandra Rosati, PhD, an assistant professor of psychology and anthropology who heads the Cognitive Evolution Group at the University of Michigan, realized the question might reveal clues about the evolution of the human mind.
“Cooking is thought to be a major evolutionary shift in the human species,” Rosati explains. According to the hypothesis proposed by Harvard University primatologist Richard Wrangham, PhD, embracing a cooked diet allowed our pre-Homo sapiens human ancestors to extract more energy from food, thereby growing bigger brains and evolving into the species we are today. But that hypothesis hinges on the idea that cooking was adopted early in human evolution—a theory that remains contested. “We thought that psychology could bring a new line of evidence to this evolutionary idea,” Rosati says.
Cooking is a complex behavior that requires multiple cognitive abilities, including the preference for cooked food over raw, the patience to wait for food to cook, a causal understanding that a cooking device can transform raw food into cooked, the willingness to hand over valued food with the expectation that it will return in a more delicious form later and the ability to plan ahead by saving raw food to cook later instead of eating it right away. With her University of Michigan psychology colleague Felix Warneken, PhD, Rosati performed a series of experiments using a homemade faux chimpanzee “microwave,” a covered bowl in which researchers could surreptitiously swap slices of raw potato for cooked potato. They found the chimps demonstrated all the cookingrelated cognitive abilities they tested ( Proceedings of the Royal Society B , Vol. 282, No. 1809, 2015).
“If chimps can do it, our early human ancestors probably could do these things, too,” Rosati says—a finding that adds another piece of support to Wrangham’s cooking hypothesis. “This experiment lets us see how a set of psychological abilities can, potentially, let an animal move into a new evolutionary niche,” she adds.
Rosati’s research is part anthropology and part evolutionary biology. But her lab is situated in the psychology department, and she couldn’t tackle existential questions about the evolution of human thinking without leaning heavily on psychological science. “My overarching research goal is to understand where complex human cognition comes from, and more generally, how cognition evolves at all,” she says. “By combining ideas from psychology, about how the mind works, with ideas from anthropology, about what the mind is for, we can come up with entirely new things to study—things that perhaps nobody would have thought of otherwise.”
Lemurs, apes and humans
Rosati has an undergraduate degree in psychology from Harvard University and earned her PhD in evolutionary anthropology, with a certificate in cognitive neuroscience, from Duke University in 2012. She spent two years as an assistant professor at Harvard before moving her lab to Michigan in 2017. As a fairly new arrival, she’s still growing her lab—she currently advises two graduate students and one postdoc.
“People sometimes wonder why we do this work with animals. But it’s a unique opportunity to explore how other minds work, how they see the world and what that can tell us about the evolution of the human mind,” says second-year PhD student Averill Cantwell.
Rosati’s team takes a comparative approach, studying a variety of primate species from lemurs (the most primitive of the living primates, and the most distantly related genetically to humans) to apes (our closest living genetic cousins in the animal kingdom). Rather than keep animals on campus, Rosati and her students travel to them, studying free-ranging primate populations in sanctuaries, parks and research centers in the United States, Europe and Africa. That model allows them to study a more diverse range of animals in situations that mimic their natural physical and social environments. “That’s important, since we want to understand how cognition works in complex real-world environments,” Rosati says.

In fact, many of her methods borrow from developmental psychology. Like human babies, chimps and monkeys can’t fill out surveys or answer questions. Instead, the researchers rely on methods such as gaze-following to track an animal’s attention and using looking time as a marker of surprise.
Making decisions
One theme in Rosati’s research is studying how and why animals make the decisions they do. The research is inspired, in part, by findings from behavioral economics that show humans make some strange choices. We favor short-term consumption over long-term investments, for example, and arbitrarily assign greater value to products based on popularity and status. Our primate cousins can help us better understand those quirks. “Animals don’t use money or credit cards, but they do have to make a lot of decisions about value when foraging for food or looking for mates,” Rosati says. “We’ve been exploring the hypothesis that even though some of our human biases look irrational from an economic perspective, they might actually be quite rational from a biological perspective.”
In one example, she compared chimpanzees with their sister species, bonobos. Though similar in many ways, the two apes live very different lives. Chimps have evolved to feed on highercalorie foods that are more difficult to come by: hard-to-find fruits, nuts that require cracking and ants that they must “fish” for with sticks inserted into the ants’ nests. Bonobos eat fruit but rely more heavily on plentiful vegetation, and unlike chimps, they’ve never been seen using tools in the wild. Those differences play out in the two species’ decision-making behaviors, as Rosati described in a recent review article ( Trends in Cognitive Sciences , Vol. 21, No. 9, 2017). Chimps, she found, are more patient than bonobos, have better spatial memory and are more willing to take big risks in hopes of big payouts. What looks irrational in one environment seems perfectly logical in another.
What about us? People, Rosati found, tend to look more like chimps in their risk-taking behavior—suggesting that our species might have evolved in an environment similar to the one chimps evolved in, she says. Indeed, human hunter-gatherers today often forage for high-risk, high-reward food (such as hunting for meat and gathering honey). Yet Rosati also found that while humans are chimplike when making decisions about food rewards or prizes, they’re more risk averse when money is at stake. That suggests there might be something evolutionarily novel about money, she says—and that different psychological processes might be involved when we’re thinking about concrete versus abstract rewards ( Evolution and Human Behavior , Vol. 37, No. 2, 2016). It’s a finding that could have implications for anyone who studies behavioral economics. “Studies that use money as a reward might not be capturing the full picture of how humans make decisions,” she says.
Friends and fruits
It might seem obvious that an animal’s physical environment would influence its evolutionary path. Yet much research on primate and human cognition has focused on the role of living in complex social groups, Rosati says. To fully understand how the human mind came to be, she argues, scientists should consider how social and ecological pressures work in tandem.
Lemurs make excellent subjects for that, says Francesca De Petrillo, PhD, a postdoctoral researcher of Rosati’s who is now based at the Institute for Advanced Study in Toulouse, France. All types of lemurs share a single common ancestor, but some lemur species live in complex social groups, while others have much looser social affiliations. Some graze on readily available leaves, while others eat fruit, a more cognitively demanding food source that requires finding fruits, determining whether they’re ripe and, if not, waiting until they’re ready to pick.
In one ongoing project based at the Duke Lemur Center at Duke University, De Petrillo is comparing the leaf-eating Coquerel’s sifaka lemur with the fruit-eating red ruffed lemur to determine whether each species is capable of logical inference—that is, if they can use information they’re given about where food is located to deduce new information about the location of hidden foods. “We want to understand how ecology, and especially diet, may have shaped their cognition,” she says.
In other work, Rosati’s team is zeroing in on the social side of the equation. In a number of studies, she has compared a population of free-ranging rhesus macaques in Cayo Santiago, Puerto Rico, with Barbary macaques living in a forested park in the United Kingdom. Rhesus macaques are aggressive and despotic, Rosati says. Closely related Barbary macaques are much more socially tolerant and generally have a calmer temperament.
Rosati and Laurie Santos, PhD, a professor of psychology at Yale University, compared the two species to test how their divergent social systems might have influenced their social cognitive skills. They found that as juveniles, both species engage in similar rates of gaze following—turning to look at the things other members of their troops are looking at. Barbary macaques continue that behavior into old age. But by adulthood, rhesus macaques engage in gazefollowing much less often ( Animal Behaviour , Vol. 130, 2017). “The more tolerant Barbary macaques remain interested in these social cues over their life span. But for the intolerant rhesus macaques, once they reach maturity, that’s not in their bag of tricks anymore,” Rosati says.
Living long and living well
Looking across the life span is another recurring theme in Rosati’s work. One common way to understand human cognition is to explore how it develops in infants and children, she notes. Another is to look to our primate cousins to understand the evolutionary roots of cognition. Rosati wants to do both. “We’re combining those approaches to look at how cognition, behavior and physiology change across the life span,” Rosati says.
Even without modern medicine, humans can easily live past 70. Wild chimps tend to die by age 50, and macaques live just half that long. “Why is it that we’re living so long, and what can that tell us about human cognition and behavior?” Rosati asks.
In one new project, she and her colleagues are studying aging in chimpanzees. Partnering with scientists who study wild chimps, they are drawing from socioemotional selectivity theory—a psychological theory that maintains that as humans age, they hone their social networks and invest in more emotionally meaningful relationships and goals—to predict how wild chimps might behave over their life spans. “We’re trying to bridge the kinds of controlled experiments we’re doing in these sanctuaries and parks with the kinds of observational techniques that are used in the wild,” she says.
The chimpanzee work is funded by the National Institute on Aging, while other projects are largely supported by the National Science Foundation. And in 2019, Rosati was awarded a prestigious Sloan Research Fellowship in neuroscience, a two-year, $70,000 award that is helping her to dig more deeply into the origins of complex thinking.
Rosati is driven to answer fundamental questions about the evolution of cognition. She’s also thankful that her work allows her to support animal welfare. The sanctuaries where she does much of her research care for animals orphaned by hunting and the pet trade, and she serves as a technical adviser to the Pan African Sanctuary Alliance, an association of wildlife sanctuaries across 13 African nations. “Through our research, we’re able to support organizations that are playing a crucial role in primate conservation and law enforcement,” she says. “It’s a privilege to study these animals, and we need to put their welfare and well-being first.”
Further reading
The Evolutionary Roots of Human Decision Making Santos, L.R., & Rosati, A.G. Annual Review of Psychology 2015
Chimpanzee Cooperation Is Fast and Independent From Self-Control Rosati, A.G., et al. Psychological Science 2018
Ecological Rationality: Convergent Decision-Making in Apes and Capuchins De Petrillo, F., & Rosati, A.G. Behavioural Processes 2019
Flexible Gaze-Following in Rhesus Monkeys Bettle, R., & Rosati, A.G. Animal Cognition 2019
“Lab Work” illuminates the work of psychologists in research labs. To read previous installments, go to www.apa.org/monitor/digital and search for “Lab Work.”
Recommended Reading
Research foci.
The Cognitive Evolution Group is:
- Exploring how variation in cognitive abilities relates to different species’ natural history.
- Studying cognitive development and aging in monkeys and apes to understand human evolution.
- Researching the cognitive skills primates use to make decisions in their environments.
- Investigating how a species’ social context shapes decision-making.
Contact APA
You may also like.

Science, Medicine, and Animals (1991)
Chapter: why are animals used to study the brain, why are animals used to study the brain.
Just as animals are used as models to study the heart or lungs of human beings, so they are used as models to gain insight into the human brain and nervous system. But the brain is much more complex and subtle than any other organ. Not only does it control the functions of the rest of the body but it is responsible for movement, communication, memory, perception, emotion—all of the activities encompassed by the general term behavior.
It may seem paradoxical to use animals to study the one organ that most clearly distinguishes humans from animals. Animals, after all, are not capable of many of the more complex functions found in humans, such as advanced language, moral reasoning, or complex learning skills. But many of the basic structures and functions of the brain are common to all animals. Since complex human thoughts are built on a foundation of simpler mental processes that are evident in animals, animal studies can shed light on uniquely human behaviors.
Animal research involving the brain has already produced dramatic improvements in human health and well being, and it promises many more. 18 Drug therapies developed in part in animals have revolutionized the treatment of mental illness in the last generation. For example, chlorpromazine, which was developed in the 1950s using animals, and other antipsychotic drugs have eliminated the horrific conditions that used to exist in the wards of mental hospitals where the most severely disturbed patients were once kept. More generally, drugs have proven effective in treating the symptoms of schizophrenia, which affects millions of Americans. Anti-anxiety drugs that have proven of immense benefit in treating human anxiety are developed and tested with animals. Animal tranquilizers have also made it possible to examine and treat livestock, pets, or wildlife that are normally difficult to handle.
In addition, many purely behavioral treatments that do not rely on drugs have been developed through animal research. Behavior modification therapies are the most effective and widely applied techniques for treating alcoholism, drug abuse, obesity, neuroses, compulsions, and phobias. These therapies are partly the result of many years of basic animal research on the fundamental properties of learning and on the effects of reinforcement (either positive or negative) on behavior.
Animals are used in many other kinds of behavioral studies. Animal experiments have produced valuable information on the effects of visual stimulation on brain development, biofeedback techniques, memory loss, programmed instruction in education, aggression, stress, and recovery after strokes or brain injury. We would know much less about these aspects of human life without animal research, and continued animal research is essential if new ways are to be found to cope with behavioral problems. Nonhuman primates are particularly valuable in behavioral research, as they are in a wide range of biomedical research, because of their many similarities to humans. 19
|
The necessity for animal use in biomedical research is a hotly debated topic in classrooms throughout the country. Frequently teachers and students do not have access to balanced, factual material to foster an informed discussion on the topic. This colorful, 50-page booklet is designed to educate teenagers about the role of animal research in combating disease, past and present; the perspective of animal use within the whole spectrum of biomedical research; the regulations and oversight that govern animal research; and the continuing efforts to use animals more efficiently and humanely.
READ FREE ONLINE
Welcome to OpenBook!
You're looking at OpenBook, NAP.edu's online reading room since 1999. Based on feedback from you, our users, we've made some improvements that make it easier than ever to read thousands of publications on our website.
Do you want to take a quick tour of the OpenBook's features?
Show this book's table of contents , where you can jump to any chapter by name.
...or use these buttons to go back to the previous chapter or skip to the next one.
Jump up to the previous page or down to the next one. Also, you can type in a page number and press Enter to go directly to that page in the book.
Switch between the Original Pages , where you can read the report as it appeared in print, and Text Pages for the web version, where you can highlight and search the text.
To search the entire text of this book, type in your search term here and press Enter .
Share a link to this book page on your preferred social network or via email.
View our suggested citation for this chapter.
Ready to take your reading offline? Click here to buy this book in print or download it as a free PDF, if available.
Get Email Updates
Do you enjoy reading reports from the Academies online for free ? Sign up for email notifications and we'll let you know about new publications in your areas of interest when they're released.

An official website of the United States government
The .gov means it’s official. Federal government websites often end in .gov or .mil. Before sharing sensitive information, make sure you’re on a federal government site.
The site is secure. The https:// ensures that you are connecting to the official website and that any information you provide is encrypted and transmitted securely.
- Publications
- Account settings
Preview improvements coming to the PMC website in October 2024. Learn More or Try it out now .
- Advanced Search
- Journal List

Neuroethics and Animals: Report and Recommendations From the University of Pennsylvania Animal Research Neuroethics Workshop
Growing awareness of the ethical implications of neuroscience in the early years of the 21st century led to the emergence of the new academic field of “neuroethics,” which studies the ethical implications of developments in the neurosciences. However, despite the acceleration and evolution of neuroscience research on nonhuman animals, the unique ethical issues connected with neuroscience research involving nonhuman animals remain underdiscussed. This is a significant oversight given the central place of animal models in neuroscience. To respond to these concerns, the Center for Neuroscience and Society and the Center for the Interaction of Animals and Society at the University of Pennsylvania hosted a workshop on the “Neuroethics of Animal Research” in Philadelphia, Pennsylvania. At the workshop, expert speakers and attendees discussed ethical issues arising from neuroscience research involving nonhuman animals, including the use of animal models in the study of pain and psychiatric conditions, animal brain-machine interfaces, animal–animal chimeras, cerebral organoids, and the relevance of neuroscience to debates about personhood. This paper highlights important emerging ethical issues based on the discussions at the workshop. This paper includes recommendations for research in the United States from the authors based on the discussions at the workshop, loosely following the format of the 2 Gray Matters reports on neuroethics published by the Presidential Commission for the Study of Bioethical Issues.
INTRODUCTION
Growing awareness of the ethical implications of neuroscience in the early years of the 21st century led to the emergence of the new academic field of “neuroethics.” Academic meetings and symposia, new journals, emerging university centers and programs, and an official society have provided venues for discussion of neuroethical issues. The importance of this new field was demonstrated in 2013, when President Obama announced the launch of the National Institutes of Health (NIH) BRAIN Initiative created with a mission of revolutionizing our understanding of the brain and simultaneously called on the Presidential Commission for the Study of Bioethical Issues to create a working group to specifically address the complicated ethical issues that arise from our rapidly increasing neuroscientific knowledge.
Two volumes entitled Gray Matters resulted from this examination of neuroethical issues by the working group. The first volume, released in May of 2014, examined “the integration of ethics into neuroscience research across the life of a research endeavour.” 1 The second volume, released in March of 2015, focused on “a set of controversial topics that illustrate the ethical tensions and societal implications of advancing neuroscience and technology: cognitive enhancement, consent capacity, and neuroscience and the legal system.” 2 The 2 volumes of the Presidential Commission for the Study of Bioethical Issues report surveyed a broad and complex landscape of ethical issues, presented a concise set of illustrative examples, provided insightful analysis, and included concrete, practical recommendations throughout. The reports have provided and will continue to provide valuable guidance for neuroscience researchers and policymakers for years to come. As an example, the Journal of Neuroscience recently published a set of 8 guiding principles for the BRAIN Initiative from the NIH Neuroethics Working Group along with an accompanying commentary. 3 , 4
The vast majority of initial BRAIN Initiative funding was targeted at developing animal models of brain function, and the NIH recently announced a call for applications for creating new colonies of marmosets for “transgenic and chimeric neuroscience research.” 5 But despite the rapid acceleration and evolution of neuroscience research on nonhuman animals, neither the 2 Gray Matters volumes nor the recent 8 guiding principles make any reference to ethical issues connected with neuroscience research involving nonhuman animals. This is a significant oversight given the central place of animal models in neuroscience. As such, there is a strong need for exploration of neuroethical issues involving animals.
Though several reports and guidance documents exist that are focused on the use of animals in neuroscience research, these documents have primarily focused on applying familiar ethical frameworks (such as the “3Rs” of reduction, replacement, and refinement) to neuroscience research rather than emphasizing new ethical challenges unique to neuroscience. But just as it has been a central theme from neuroethics that our increased abilities to interpret and influence human brains have given rise to unique ethical questions that differ from traditional questions in bioethics, 6 our increased ability to study and manipulate animal brains also raises new questions and concerns that go beyond previous discussions of animal ethics. These new questions have not yet been addressed in a systematic and comprehensive manner, though the need for neuroethics to include explicit discussion of issues involving nonhuman animals is increasingly being recognized in special journal issues, 7 statements from journal editors, 8 and edited volumes. 9
To respond to these concerns, the Center for Neuroscience and Society (CNS) and the Center for the Interaction of Animals and Society at the University of Pennsylvania hosted a workshop on the “Neuroethics of Animal Research” in Philadelphia, Pennsylvania, in June 2016. This workshop featured expert speakers and participants in neuroscience, ethics, psychology, veterinary medicine, and epidemiology as well as representatives from the NIH BRAIN Workgroup, the Committee on Guidelines for the Care and Use of Mammals in Neuroscience and Behavioral Research, the pharmaceutical industry, and an animal protection organization. The workshop was focused on identifying emerging and under-discussed ethical questions arising from neuroscience research involving nonhuman animals and was organized by Martha Farah, Director of the CNS, Adam Shriver, Visiting Research Fellow at the CNS, and James Serpell, Director of the Center for the Interaction of Animals and Society.
After brief introductory and stage-setting remarks from Martha Farah and Adam Shriver, the 1-day workshop focused on 5 topics presenting new ethical questions. The morning session was focused on the use of animal models in neuroscience research on pain and psychiatric conditions and featured presentations from Kenneth Sufka, Professor of Psychiatry at the University of Mississippi, Joseph Garner, Associate Professor of Comparative Medicine at Stanford, and Larry Carbone, Director of the Animal Care and Use Committee at UCSF. The afternoon was focused on “ontology-bending” new technologies that challenged our previous conceptions of “human,” “animal,” “person,” and “machine.” This session featured presentations from Andy Schwartz, Professor of Neurobiology at Pittsburgh, Evan Ballaban, Professor of Psychology at McGill University, Helena Hogberg, Research Associate at Johns Hopkins University, Robert Streiffer, Professor of Bioethics and Ethical Theory at Wisconsin, and Kristin Andrews, the York University Research Chair in Animal Minds. General discussion was included throughout the day’s presentations.
This report provides an overview of the ethical issues that arise in neuroscience research on nonhuman animals based on the workshop presentations and discussion. This includes a discussion of the use of animal models of pain and human psychiatric disorders, an analysis of recent technological breakthroughs relating to neuroscience research on nonhuman animals, and an overview of the ways that neuroscience interventions can challenge traditional categories of moral patienthood. Based on these discussions, we include analysis here of key ethical questions related to neuroscience research on nonhuman animals. From these discussions, we (the authors of this paper) propose recommendations for ethical conduct of neuroscience research on nonhuman animals moving forward. These recommendations should be considered the authors’ own and may not represent the views of other workshop participants or that of the funding or hosting institutions. Though the workshop was several years ago, the topics covered continue to be important ethical issues.
ANIMAL MODELS OF PAIN AND PSYCHIATRIC CONDITIONS
Neuroscience research has progressed tremendously over the past half century, but has also seen serious and expensive failings in attempts to use animal models of human mental states such as pain 10 as well as animal models of treatments for psychiatric conditions. 11 These topics were discussed at the workshop by Drs Kenneth Sufka, Joseph Garner, and Larry Carbone.
Regarding pain, many of the most common assays used in pain research on nonhuman animals (such as hot plate, Von Frey fibers, and tail flick tests) produce observable reactions that are spinally mediated and thus do not depend on brain activation. Pain is defined as having sensory and affective components, 12 and the affective component of pain is arguably the most clinically significant, 13 yet the most common tests in animals do not capture affect. Moreover, most assays are testing acute pain, but in humans the most serious clinical challenges involve chronic pain. 14 As Kenneth Sufka stated succinctly during his presentation, “I have yet to figure out why we need to develop an analgesic for humans who leave their hands on a hot stove for too long.” Moreover, even when innovative new models of chronic pain are developed, they are often “validated” using the same measures of pain that are spinally mediated rather than brain dependent. A recent review explains that “[f]rustration is mounting over the limited success of the field in translating the veritable explosion of basic scientific data collected over the past few decades using animal models into truly new, effective and safe clinical analgesics.” 10
For this reason, Sufka 15 , 16 and others 17–19 have developed conditioned place preference measures of pain. In these tests, animals are exposed to different stimuli in specific locations, and their willingness to approach or avoid those locations in the future is measured. Conditioned place aversion appears to rely on activity in the brain regions that are crucial for pain affect and chronic pain symptoms in humans. Moreover, the animals choosing to avoid areas they associate with negative events intuitively seems to better capture the negative valence of pain that makes pain undesirable for humans. The facial grimace scale 20 also appears to be a promising method for evaluating pain affect in mice. Nevertheless, much pain research still uses older models that do not appear to capture the most clinically significant aspects of pain, rendering them poor predictors of analgesic efficacy. The primary purpose of pain research on animals is to find effective treatments of pain in humans, but most effective analgesics are still “based on mechanisms of action that have been recognized for some time,” and many new compounds designed as painkillers are failing clinical trials. 21 As stated in a recent review, “Many are frustrated with the lack of translational progress in the pain field, in which huge gains in basic science knowledge obtained using animal models have not led to the development of many new clinically effective compounds.” 10 This failure has only become more significant as the opioid crisis continues to cause massive harms due, in part, to the inability to find effective analgesics with less addictive properties than opioids.
As explained by Joseph Garner at the workshop, there are arguably even more severe translation problems when it comes to the modeling of human psychiatric conditions and brain-based diseases, and particularly with preclinical trials of drugs intended to treat those conditions. The success rate of drugs in clinical trials that made it through preclinical testing on animals is only 1 in 10 in humans. 22 Because the primary justification given for invasive, non-therapeutic animal research is that the potential benefits outweigh the potential harms, this “90% devaluation” of the animal tests places serious pressure on the ethical justification for such research. Though the failure of human trials is not entirely a result of a failure to translate, the translation failure is nevertheless a major part of the explanation. These high failure rates not only mean that many animals are needlessly suffering but also that limited financial resources are lost.
In 2010, a major shift occurred when researchers began to question the validity of the animal models being used. One review found that of approximately 200 genetically modified mouse models of Alzheimer’s disease, none of them had been replicated in human studies. 23 Another review published the same year found that of 500 studies reporting effective treatments for ischemic strokes, only 2 successfully translated to humans. 24 Specifically in regard to animal models of human psychiatric disorders, Nestler and Hyman wrote: “Many of the symptoms used to establish psychiatric diagnoses in humans (for example, hallucinations, delusions, sadness and guilt) cannot be convincingly ascertained in animals.” 11 Researchers, and particularly the pharmaceutical industry, have started seriously considering the possibility that the animal models being used might be unreliable.
The discussion of concerns about translation models continues today. A recent proposed roadmap for Parkinson’s research stated, “Currently, no animal model faithfully reproduces all the key clinical features of PD.” 25 A valuable overview of some of the issues with the validity and reproducibility of animal models used in neuroscientific research can be found in Johnson, 26 and a more general overview of recent evidence relating to the quality, validity, and value of pre-clinical animal research regardless of scientific discipline can be found in Pound. 27
Garner has developed an extensive critique of current models. At the workshop, he argued that treatments that appear to work in animal models have a poor track record in humans in part because too many research protocols are “modeling the model” rather than “modeling the disease.” That is, they are continuing with a “model” of a disorder that is known to inaccurately capture a similar disorder in humans. Many behaviors or assays in animals taken to be models of particular disorders of humans are inadequate. For example, all of the models of Parkinson’s in animals involve dopaminergic cell loss. However, in humans, there are symptoms predictive of Parkinson’s that occur well before any dopaminergic cell loss. Thus, Garner argues, the model is only capturing a symptom, rather than an underlying cause, of the disorder. The forced-swim test is another well-known model with clear welfare issues that is a questionable “model” of multiple human psychiatric conditions 28 , 29 but is nonetheless widely used, though this may finally be starting to change. 30
Why do so many inadequate models persist in the search for human treatments? Garner offered several explanations, as follows.
The Incentive Structure of Academic Publishing. Pharmaceutical companies have started to move away from some of the least translatable animal models more quickly than academic researchers. These companies lose money from poor choices and thus are incentivized to stop using non-predictive models. However, NIH R01 grants are a central feature of the research funding structures for universities. R01 grants are renewable, and there is a 5 to 10 times greater chance of having an R01 grant renewed than getting a new R01 grant funded. Because grants are often tied to models, researchers are incentivized to continue using the same models, as they are much more likely to have grants using the same model renewed than to have new grants using different models approved. Moreover, if many of the reviewers for journals are invested in an old model for a disorder because of their own background, this can lead to problems with new models being adopted.
A Lack of Clinical Knowledge. Researchers who study models of disorders in animals do not necessarily see humans in a clinical context with that disorder. Many models are based on descriptions from the Diagnostic and Statistical Manual of Mental Disorders, but these criteria only partially and imperfectly capture the expression of disorders. Thus, a lack of experience with humans with a particular condition can lead researchers to miss cues that the model is not truly getting at the same disorder, cues that would be apparent from a more holistic understanding of the disorder in humans.
A Lack of Comparative Knowledge. Many researchers are not experts in the biology and behavior of the animals they are working with. This can lead to mistakes in interpretation or in treatment.
Excessive Homogeneity Among Research Subjects. The goal of human clinical research is to test treatments on a diverse and heterogeneous portion of the population to ensure it is an effective treatment for humans generally. However, in much animal research, the approach is dramatically different: genetically similar animals kept in identical housing with identical temperatures on identical feeding schedules are used, and this may lead to false indications that a particular treatment is effective (or ineffective) due to the focus on a narrow range of animals considered.
Ethical Issues With Animal Models
One problem raised repeatedly throughout the workshop was that the putative ethical justification for animal research is based on an assumed harm-benefit analysis where harms to nonhuman animals are outweighed by the potential benefits that would accrue to humans (or, in some cases, to other animals). Though important steps have been taken with the creation of the ARRIVE Guidelines 31 and the AALAS–FELASA Working Group reports on harm-benefit analysis, 32 at present there is no governmental requirement for performing genuine harm-benefit analysis in particular research programs in the United States. Funding agencies determine whether research is worth funding, and IACUCs are tasked with minimizing harms, but there is no requirement for directly weighing harms against expected benefits, so in fact a harm-benefit analysis is never strictly speaking required at any point in the research planning process.
Some have attempted to argue that the fact that funding agencies evaluate potential benefits and IACUCs are asked to minimize harms indicates that a reliable harm-benefit analysis does exist in the US system, but this is based on a misunderstanding of harm-benefit analysis. If the expected harms and benefits of research are never directly evaluated against one another, then there can be no basis for thinking that the benefits of research are comparatively more significant than the harms, no matter how selective funding agencies are and no matter how judicious IACUCs are. This fact is codified in the requirements of institutional research boards that oversee research on humans who are asked to both minimize risk and to ensure a favorable risk–benefit analysis. Merely minimizing risk, even where research has clear benefits, is not sufficient for ensuring a favorable ratio of benefits to risks.
Moreover, the 2015 NIH decision to eliminate most chimpanzee research on the grounds that such research was unnecessary is evidence that the current process for approving research is not robust enough to rule out animal models that are not justified by a true harm-benefit analysis. Both funding agencies and IACUCs arguably performed their roles correctly in evaluating previous chimpanzee research; nevertheless, when an independent commission looked at the research, it concluded that most of the research on chimpanzees was not necessary. Thus, despite the challenges of assessing harms and potential benefits from research, it nevertheless should be the case that there is a point in the process where the harms and expected benefits of the research are directly weighed against one another, and this analysis should be presented in a clear and transparent manner.
Nevertheless, a favorable harm-benefit analysis by no means guarantees ethical research standards. Workshop participants, bioethicists, and researchers generally were divided over what additional ethical restrictions exist on experimentation on nonhuman animals. Larry Carbone noted that many bioethicists believe that harming others without their consent is virtually always wrong and that this constraint may extend to nonhuman animals. 33 , 34 Workshop participant David DeGrazia, along with Jeff Sebo, has argued that morally responsible animal research must give each animal a worthwhile life, cause no unnecessary harms, and meet the basic needs of each animal in addition to having a favorable harm-benefit ratio, and that even satisfying these constraints may not be a guarantee of ethical research. 35
It is widely recognized that keeping animals in restrictive conditions, performing invasive procedures on them against their will, and ultimately ending their lives causes harms to those animals. Even the strongest proponents of animal research recognize these as harms but claim that those harms are justified by the potential benefits of animal research for humans. However, if thousands of animals are used in failed models lacking predictive validity, this research causes harm to animals without producing any benefits. Harming nonhuman animals without producing any benefits is not justifiable. Moreover, there are direct costs to human interests from relying on inefficient models. This money could be redirected to productive ends, whether in the pursuit of scientific advancement or on other societal priorities.
IN VITRO BRAINS (OR BRAINS-ON-A-CHIP)
Current models in toxicology testing rely on testing toxins on large numbers of animals, usually mice. These are referred to as in vivo testing and are currently considered the “gold standard” of toxicology testing by the FDA. Such research requires thousands of animals to be exposed to aversive experiences and killed. Alternative “in vitro” tests have been developed that examine the effects of toxins on cells that are not in living organisms but rather in artificial environments. 36 In an optimistic assessment at a 2017 Senate Appropriations Committee, NIH Director Francis Collins suggested that in 10 years, no animals would be used in toxicity testing and the field would move entirely to in vitro models. 37
Unfortunately, as explained by workshop participant Helena Hogberg, because the FDA treats in vivo models as their gold standard for translational research, alternative, non-animal models must typically be validated against animal models to gain federal approval. Because mouse models are imperfect models of human disease, this means that new alternatives must mirror an imperfect model to be implemented in toxicity research. On the US Food and Drug Administration (FDA)’s current regulatory standards, alternative models could in principle model human disease better than mouse models and fail to gain approval, and no model could in principle receive a stronger evaluation than a mouse model. FDA standards therefore make it politically impossible to develop models of human disease that are more accurate than mouse models. Researchers at the Center for Alternatives to Animal Testing at Johns Hopkins hope to identify toxicity pathways and mechanisms that will allow in vitro models to become the new standards for testing—thus greatly reducing the number of animals harmed—but this will require FDA endorsement and acceptance.
As part of this search for alternative and better methods of toxicology testing, researchers have been developing “organ-on-a-chip” models that can be used to assess toxicity at the molecular, cellular, and organ levels. Models have been developed for livers, eyes, and other organs. Efforts are also underway to create a “human-on-a-chip” that connects different organ models for a more holistic representation of the interaction of different systems within humans. At Johns Hopkins University’s Center for Alternatives to Animal Testing, researchers are designing models to assess neurodevelopmental toxicology by using brain-on-a-chip models. The motivation for this work is that 1 in 6 children in the United States have 1 or more developmental disorders, many of which are neurodevelopmental disorders. The National Research Council estimated that 3% of developmental disorders are due exclusively to chemical exposure and 28% are due at least partly to chemical exposure. 38
Researchers have developed a 3-dimensional partial model of rat brains that contains neurons, astrocytes, and microglia. These neurons exhibit spontaneous electrical activity, although they receive no sensory stimuli. The models trace the development of cells from induced pluripotent stem cells to neural precursor cells and finally into neurons, thus demonstrating the influence of various chemicals in the cells’ environment. The phenotypes of these cells have been shown to mimic the behavior of neurons in humans with various neurodevelopmental disorders such as Parkinson’s.
Ethical Issues With In Vitro Brains
If these models are successful, they could replace the thousands of nonhuman animals who are harmed and killed in toxicity testing each year. Animals in toxicity tests can live extremely compromised lives, experiencing acute and chronic pain due to experimentation. The benefits of this change would therefore be quantitatively and qualitatively immense and has the potential to save thousands of animals from pain, distress, and death in toxicity tests. The EPA recently decided to allocate $4.25 million to funding the research of alternatives, including $849,000 to alternative models of neurotoxicity. 39 Additional funding could accelerate this research and spare many animals from needless harm, and could help lead to the replacement of animal models as the gold standard of neurotoxicology testing.
However, one possible concern with neural arrays is whether groups of neurons, when they reach a certain level of complexity and organization, could produce sentience and therefore attain some moral status. This might initially sound implausible, but most neuroscience researchers agree that our minds and other animals’ minds ultimately consist of nothing more than coordinated neural activity, so at some point arrays of neurons organized in particular ways do attain moral status. Given the small number and limited functional connections of neurons involved in current 3D models, it seems unlikely that they presently exhibit any morally significant sentience. We should nevertheless ask if, as the models become more complex, they will eventually reach a point at which sentience is a genuine concern. Many researchers have expressed the idea that neural models raise no ethical concerns because they are not “connected to the outside world.” This can be read in 2 ways: (1) researchers might believe that, as a matter of empirical fact, when neurons are not connected to sensory inputs they fail to develop patterns of firing that are constitutive of thought or feelings; or (2) researchers might believe that a connection to the external world is required to have semantics, that is representation of the external world, in addition to syntax, a set of rules that govern how thoughts are related to one another. 40 Both of these readings, if true, may imply that neural models are not sentient and are not objects of moral concern.
Though there seems to be widespread belief that the absence of input negates the possibility of moral patient status, there are at least some reasons for caution. First, the absence of a nociceptive signal does not always entail the absence of pain. For example, phantom limb pain occurs in patients who have lost their limbs and the nociceptors that are normally responsible for pain in the limbs. In other words, pain can occur in the absence of a signal coming from the peripheral nervous system. However, unlike in the neural array models, in the case of phantom limbs, there was a previous connection to external signals, raising the possibility that the cases are not relevantly similar. Second, spontaneous patterns of activation can occur in self-contained groups of neurons. In the absence of an ability to decode neural patterns into content, we cannot decisively rule out the possibility that this organized activity has some representational content. Though some initial texts have started to consider these challenges, 41 , 42 this is an issue that requires future exploration.
ANIMAL BRAIN-MACHINE INTERFACES (BMI)
The development of BMIs has already shown tremendous potential to help humans with serious medical conditions. Human patients previously paralyzed or missing the ability to control a limb have been able to move mechanical prostheses via technology that reacts to the neural firing patterns that would normally control natural body parts. This technology has been used to help humans who have lost the ability to move a limb and has the potential to be used by patients with more severe conditions such as locked-in syndrome.
As described by workshop participant Andrew Schwartz, a key step in the development of BMI technology was using single- and multi-unit neural recordings in cortical areas associated with motor control to monitor how certain neurons fired when monkeys made arm movements. 43 After these patterns were detected and decoded, researchers inserted electrodes capable of controlling prosthetic limbs into the monkeys’ brains. The monkeys were then able to control the mechanical limbs with their brains. This research made possible current BMI in humans, and such interfaces are unlikely to have developed without these animal models given our current inability to use non-invasive technology to study the behavior of individual neurons.
BMI technology has also been put to use in controlling animal behaviors in some well-publicized cases. The “roborat” was an example of a rat whose movements were controlled by stimulation of the reward centers of the brain. 44 This technology is being tested by the US military as a way to use animals with cameras attached to them to gain information about otherwise inaccessible locations. The developers of the roborat claim that the fact that reward centers of the brain are the mechanism for guiding the rat’s behavior suggests that this is not an unpleasant experience.
However, some attendees at the meeting questioned this claim, noting that motor control disorders in humans, which cause involuntary movements, are known to be extremely aversive. This could perhaps be empirically explored in more detail by, for example, looking at cognitive bias tasks 45 or assessing facial features 46 during control tasks.
Neuron-machine technology has also been put to educational, or at least commercial, use. A group called Backyard Brains developed a “do-it-yourself kit” for creating what they call a “roboroach.” 47 Using this kit, children can dissect the antennae off of insects, then insert electrodes that can be used to control the movements of the animals. The company suggests that the roboroach teaches children about the value of science.
In addition to BMI, another class of interesting findings could perhaps be called “machine-brain interaction.” DeMarse and Dockendorf reported the use of cultured rat cortical neurons to control the flight of a simulated aircraft, 48 and Reger et al inserted a portion of a lamprey’s nervous system into a small robot as part of the control of its movements. 49 In these cases, neurons are used as necessary parts of machines.
Ethical Issues With Animal BMIs
Though there are some who question whether animal models will be needed for neuroscience research going forward, the development of BMI technologies beneficial for humans may have been unlikely without certain extensive invasive experiments on nonhuman animals. Thus, under a harm-benefit analysis of the use of animals in this type of medical research, benefits to paralyzed and immobile humans, particularly as this technology continues to evolve, can be included in the benefits column. This application of BMI is in many ways a standard case of using animal models to improve human medical conditions, and so standard epistemic and ethical considerations relevant to animal research apply.
For the military and educational applications of BMI, however, new ethical issues arise. In cases where the animals’ movements are being controlled by outside sources, questions arise as to how aversive the experience is. In humans, we would also regard the loss of liberty and autonomy resulting from external control as a significant moral concern, even for young humans who may have rudimentary agency at the level of monkeys or rats, raising similar concerns for nonhuman animals. Although the monkeys and rats used in BMI experiments are doubtlessly sentient, in the case of the roboroach, the cockroach is, on many accounts of sentience, less likely to have positive or negative experiences that resemble those of humans. Consequently, the expected harms of the research may be reduced in this regard. On the other hand, it is not clear that this provides educational value that could not be achieved through other means, so the benefits of the kit are also questionable. Moreover, one might worry that using animals this carelessly could lead to a general disregard for the welfare of animals, and so might lead indirectly to additional welfare concerns. 50
Finally, in the cases of using neural arrays to guide or influence machinery, the main concern is related to above worries about in vitro brains and the minimal conditions needed for organized neural activity to count as morally significant. Again, it seems intuitively unlikely in the above machine-brain interface cases that the neural arrays in question have anything approaching sentience, and even if the arrays did have some aspects of conscious experience there would be no reason to suppose that the phenomenology would have any positive or negative affective valence. However, an additional wrinkle compared with the in vitro neural cells is that neurons in machine-brain interfaces do have a connection to “the outside world.” That is, in contrast to claims that isolated neural arrays could have “syntax, but not semantics,” the firing patterns of these neural arrays are better candidates for representing features of the outside world. Thus, there might exist additional reasons for being cautious about their creation.
Chimeras are organisms that contain genetic material from 2 or more different sources. The chimeras of interest for neuroethics are organisms whose brains contain genetic material from multiple sources. We use “neural chimeras” to refer to such animals who have neurons from multiple genetic sources. For such chimeras, nomenclature is often used referencing “X-Y chimeras,” where X and Y refer to different types of organisms. For example, a “human-animal chimera” refers to a chimera that contains cells with a human origin and cells with a nonhuman animal origin.
Modern technology allows gene insertion to take place early in development while cells are still in the neural crest stage of development. For bird species, this means that the insertions can be performed in eggs so that no invasive surgery on sentient organisms is required. By tracking how cells from the neural crest develop into brain cells, researchers can selectively intervene such that cells in particular regions of the developed brain originate exclusively from the donor organism while cells in other brain regions originate from the host embryo. Thus, targeted precision for the combination of neural material is possible.
At the workshop, Evan Balaban provided an example of the power of neural chimeras for demonstrating the role of neurons in behavior using his research. 51 Male chickens and quail each have their own characteristic vocalizations. In separate experimental arms, Balaban and colleagues inserted quail cells into the cellular progenitors of neurons that compose the midbrain, the brainstem, and the forebrain of chicken embryos, respectively. In the condition where quail neurons were in the midbrain, but not the other 2 conditions, the resulting chicken-quail neural chimeras made characteristic quail vocalizations along with the characteristic quail head bob. This research demonstrated both the utility of neural chimeras to produce behavioral changes and a technique for using neural chimeras to investigate mechanisms. Such techniques could be used to further investigate the role of neurons in behavior.
In another study, mice with a human FOXP2 gene made slightly different vocalizations and showed subtle anatomical and physiological differences in brain regions used for speech and other movement. 52 This shows that a human gene can influence brain development in a mouse and exert behavioral effects. Although single genes rarely cause large effects by themselves, other methods of merging animal brains with larger-scale functional elements, either biological or electronic, could produce significant psychological changes, increasing an organism’s cognitive capacities.
The possibility of “humanizing” other animals has been the subject of much controversy; the NIH has a ban on human and nonhuman chimeras in place but has proposed lifting this ban. However, there are currently no special restrictions on animal–animal chimeras.
Ethical Issues With Chimeras
Inserting cells from one organism into another host organism has the potential to alter the cognitive and affective capacities of the host animal. It seems very likely that as technology becomes more sophisticated, this technique could increase the intelligence of organisms and could alter their capacity for positive and negative feelings. Because the cognitive life of an organism and the species of an organism are often tied to their moral status, the moral status of a neural chimera—and correspondingly the wrongness of subjecting that chimera to involuntary experimentation, suffering, and death that would follow from this status—cannot be assumed to be equivalent to the moral status of a typical adult member of the host species.
If we combine neural cells from 2 different organisms to create a neural chimera, this raises the question: what ethical standards should we apply to the new organism? Should we apply the standards we would normally hold for the more protected of the 2 organisms? If so, in cases of nonprimate-primate chimeras, current regulations would require that we account for the psychological well-being of the chimera, because this is what is currently required for the care of primates. And in cases of human-animal chimeras, current regulations would place a very high standard on such research that could even require consent, making the permissibility of such research unlikely. The human-mouse chimera discussed above, for example, would require full institutional review board approval applying all of their ordinary standards for human subjects research.
Of course, inserting a small number of human neurons into a nonhuman animal is unlikely to transform the cognition of the animal in any significant way, so a more sophisticated approach would not apply blanket ethical rules to all chimeras based on the species of origin but would instead attempt to assess the moral significance of each alteration. This leads to difficult questions about how to assess the moral status of the created being. It should be noted that although there has been much discussion of human-animal chimeras in the bioethics literature already, animal–animal chimeras raise related but underexplored questions about the moral status of the new organism.
The ethicist Robert Streiffer argued at the workshop that the moral status of neural chimeras depends entirely on that chimera’s cognitive capacities. If a neural chimera has the cognitive capacities of an adult chimpanzee, then that animal has the same moral status as an adult chimpanzee. If they have the cognitive capacities of an adult beagle, then they have the same moral status as an adult beagle. Such an account is revisionary for animal research guidelines, for it implies that organisms who have the same cognitive capacities should have the same protections. Thus, organisms who have the cognitive capacities of young humans (perhaps including mice) should have the same protections as young humans. Streiffer’s argument parallels an approach that has significant agreement in the animal ethics literature and claims the following: the species of an animal is not morally significant, and animals who are the same in virtually every way except for species membership have equal moral status.
Previous discussions of animal research neuroethics have focused on how new technologies in the neurosciences open up new and interesting ethical questions. However, a different set of questions in neuroethics focuses on how new knowledge from the neurosciences might inform existing ethical debates. For example, the role of neuroscience in determining the sentience of various species has been discussed extensively in the literature. At our workshop, we chose to focus on how neuroscience might inform a different set of morally salient characteristics: those making up the concept of “personhood.”
As noted by the first speaker, Kristin Andrews, there are various different senses of “personhood” that are sometimes confused in debates on the topic. In the law, personhood signifies legal standing and is contrasted exclusively with “mere things.” 53 Thus, in the law, lawsuits can be filed on behalf of a person whose rights have been violated. However, anything the law classifies as a mere thing, including, at the present moment, all nonhuman animals, has no such standing and, in fact, has no more legal standing than inanimate objects. Some advocacy groups, such as the Nonhuman Rights Project, are currently working to see courts recognize that some nonhuman animals can meet the legal standards for personhood. 54
In contrast, psychological personhood refers to a suite of psychological properties that provide the basis for suggesting that a given entity has a cognitively sophisticated point of view. These properties can include such capacities as metacognition, a sense of self, moral behavior, and linguistic capacities, but in general there is not widespread agreement as to what the characteristics of psychological personhood should be.
Finally, the notion of most direct relevance for our workshop is the notion of moral personhood, which refers to having a particular moral status that is worthy of a suite of protections that generally go beyond the protections we provide for the “merely sentient.” The moral notion of personhood is sometimes, but not always, linked to the notion of moral agency; on this conception, to be a person means to be a being capable of acting on moral considerations, and this ability is suggested to make the being worthy of an extra level of moral considerability.
As detailed by Andrews, the set of capacities that make up the psychological notion of personhood, which often informs the moral notion, can be divided into different categories. These categories include rationality, autonomy, subjectivity, personality, a narrative sense of self, and relationships with others. It is presumably for these specific domains, rather than for personhood considered as a whole, that neuroscience could be most relevant. There are no conclusive findings yet, but future neuroscience may inform debates about whether nonhuman animals have person-like capacities. For example, it might turn out that spindle cells enable certain advanced forms of cognition, in which case they might be thought of as biomarkers for those functions. Stanislas Dehaene has argued that certain features of human neural organization have given rise to the capacity for more abstract thought. And certainly those notions of personhood that favor language capacity are likely to find particular properties of neural organization that uniquely support this capacity.
In general, however, most attendees of the workshop agreed that the notion of “personhood” itself was not especially helpful for most moral debates concerning the use of animals in research. Focusing on personhood in the legal context makes some sense given that the law is highly dependent on precedent and that according to current precedent, “persons” and “things” are logically exhaustive categories. However, in the moral context, it is likely that specific traits associated with personhood, rather than the combined cluster of traits that are vaguely referred to as “personhood,” will be most relevant for ethical questions. For example, whether there should be special provisions for social housing or interaction seems to depend primarily on an organism’s level of social attachment rather than on broader questions of the organism’s “personhood.” Similarly, whether an organism has a broader suite of possible interests and desires made possible by a sense of self or by autonoetic consciousness seems to be a question that does not turn on particular definitions of personhood. Thus, workshop participants considered the individual capacities suggested making up the notion of personhood as important and worthy of neuroscientific investigation, but the relevance of personhood itself was questioned.
Ethical Issues Regarding Personhood
The neural basis of specific attributes that could make nonhuman animals worthy of consideration beyond that of “mere sentience” are currently unclear. This includes the capacity for language, autonoetic consciousness, episodic memory, metacognition, assent, consent, dissent, and complex social relationships. Discussions of personhood are at present unlikely to specifically inform or guide our research guidelines, but many of the capacities associated with personhood can provide valuable insights into moral questions.
RECOMMENDATIONS
The workshop raised a number of significant ethical issues arising due to advances in animal neuroscience research. As the authors of this report, our primary aim was to summarize the deliberations of the workshop. However, an additional contribution we make is to include policy recommendations for the United States that aim at resolving some of the ethical issues discussed at the workshop. These recommendations should not be confused with recommendations arising out of the workshop itself. Although workshop participants largely agreed about the ethical issues arising, the recommendations themselves were not discussed at the workshop and are the opinions of the authors alone.
Recommendation 1: Develop Guidelines for Evaluating Animal Models of Psychiatric Conditions
This panel raised serious concerns about the reliability of current animal models of pain and psychiatric disorders. Given that the tests that produce models of these conditions are almost assuredly aversive, this raises the possibility that current paradigms are causing a significant amount of unnecessary harm to nonhuman animals. The critique of animal models from Garner and other researchers is not without controversy but raises serious issues that should be systematically addressed. We recommend that a panel of experts be convened through a well-respected organization, such as a Presidential Bioethics Commission, the National Academy of Medicine, or the National Academy of Sciences, that critically evaluates current methods used for modeling human psychiatric conditions in animals. As part of the report, a conference or workshop should be convened that represents proponents of some of the strongest critiques of these animal models as well as those who can argue ably on behalf of current procedures. In contrast to some recent panels convened by the NIH, it is critical that any such panel include significant representation from professional ethicists who can clearly articulate and fairly represent different positions in contemporary ethical debate.
Recommendation 2: Allocate Greater Funding to in Vitro Neurological Models
More government funding resources should be devoted to developing 3D models of neural function with an aim of replacing animal models of toxicity as the gold standard. This has the potential to save thousands of animals from pain, distress, and death in toxicity tests. Moreover, it might be possible for sophisticated neural models to replace animal testing in other domains as well. We applaud the EPA’s recent decision to allocate $4.25 million to funding the research of alternatives, including $849,000 to alternative models of neurotoxicity, and recommend that government funding for these initiatives continue to be greatly increased.
As part of this effort, funding should be provided to shift away from treating animal models as the gold standard of neurotoxicology testing. Hogberg’s presentation revealed a federal regulatory shortcoming: the FDA evaluates alternative toxicity tests by their degree of conformity to existing mouse models. Because mouse models are imperfect models of human disease, this limits the potential for developing disease models that are as or more accurate models of human disease than mouse models but that are less accurate models of mouse disease than mouse models. The FDA and other existing regulatory bodies should replace animal models as their gold standard with other biomarkers. Because many of these models are still in development, mouse models cannot be replaced overnight. If FDA regulatory policy is ahead of the development of such models, however, they can ensure that promising new models are evaluated by their degree of conformity to human disease rather than their degree of conformity to disease manifestations in mice and incentivize researchers to produce models of disease relying on human biomarkers. We support current efforts by the Interagency Coordinating Committee on the Validation of Alternative Methods and hope they are continued and accelerated.
Recommendation 3: Conduct Welfare Research on the External Control of Animals
Research should be conducted to assess whether external control of animals’ behavior leads to aversive states. This could be performed by, for example, looking at cognitive bias tasks or assessing facial features, but in general a variety of assessments should be used to ensure that any possible effect is noticed. If it is found that external control does have an influence on the welfare of animals, this needs to be taken into account in assessments of the harms and benefits of similar research in the future. Moreover, because we can be relatively confident that at least some forms of external control of behavior could be extremely aversive, guidelines should be established that place limits on the forms of external control that are permitted. For example, guidelines could permit only reward circuitry—rather than punishment circuitry—for controlling behavior in vertebrates.
As with all pain research, pain researchers should not conduct this research by performing independent, potentially aversive interventions where it is feasible to instead (1) measure indicators of discomfort on interventions that would already have been conducted for other experimental purposes, or (2) measure indicators of discomfort on nonhuman patients who are already experiencing potentially aversive external behavioral control. We think that often at least 1 of these 2 options is feasible.
Participants at the “Neuroethics of Animal Research” workshop from the Center for Neuroscience and Society and the Center for the Interaction of Animals and Society at the University of Pennsylvania raised significant ethical questions about new research rendered possible by advances in neuroscience. Herein, we have distilled these concerns and presented some discussion points relevant to decisions about how to improve the ethical standards of neuroscience research in nonhuman animals.
Technology is accelerating our ability to study and manipulate the brains of animals, and these new manipulations raise new ethical questions. More so than in the case of humans, cyborg and chimeric animals are not just thought experiments for the future; they have already arrived. At the same time, all signs indicate that societal concern for animal welfare is increasing. This can be seen in the NIH’s decision to phase out chimpanzee research and in numerous agricultural companies’ decisions to shift away from the most intensive confinement conditions. Significantly, recent polling indicates that concerns about the use of animals in invasive research are growing. It is important to ensure that the advancement of neuroscience is responsive to public concerns about the welfare of nonhuman animals. This calls for the constant improvement of research standards and the termination of research that is demonstrated to be unethical as well as the continuous monitoring of new ethical issues that arise from neuroscientific advances. The Penn Neuroethics of Animal Research Workshop has yielded findings that should be used toward these ethically urgent ends.
ACKNOWLEDGMENTS
The workshop was made possible by grants from the Alternatives Research Development Foundation (ARDF) and the University of Pennsylvania School of Arts and Sciences Conference Support Grant.
Potential conflicts of interest
All authors: No reported conflicts.
Animal Models for Brain Research
- First Online: 30 September 2020
Cite this chapter
- Debby Van Dam 6 , 7 &
- Peter Paul De Deyn 6 , 7 , 8
1147 Accesses
Animal models are important experimental tools in neuroscience research since they allow appraisal of selected and specific brain pathogenesis-related questions—often not easily accessible in human patients—in a temporal and spatial pattern. Translational research based on valid animal models may aid in alleviating some of the unmet needs in the current pharmaceutical market. Of primary concern to a neuroscience researcher is the selection of the most relevant animal model to achieve pursued research goals. Researchers are challenged to develop models that recapitulate the disorder in question, but are quite often confronted with the choice between models that reproduce cardinal pathological features of the disorders caused by mechanisms that may not necessarily occur in the patients versus models that are based on known aetiological mechanisms that may not reproduce all clinical features. Besides offering some general concepts concerning the relevance, validity and generalisation of animal models for brain disorders, this chapter focuses in detail on animal models of brain disease, in particular schizophrenia models as examples of animal models of psychiatric disorders and Alzheimer’s disease models as examples of animal models of neurological/neurodegenerative disorders.
This is a preview of subscription content, log in via an institution to check access.
Access this chapter
Subscribe and save.
- Get 10 units per month
- Download Article/Chapter or eBook
- 1 Unit = 1 Article or 1 Chapter
- Cancel anytime
- Available as PDF
- Read on any device
- Instant download
- Own it forever
- Available as EPUB and PDF
- Compact, lightweight edition
- Dispatched in 3 to 5 business days
- Free shipping worldwide - see info
- Durable hardcover edition
Tax calculation will be finalised at checkout
Purchases are for personal use only
Institutional subscriptions
Similar content being viewed by others
The Trouble with Animal Models in Brain Research
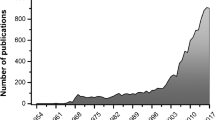
Animal models of depression: pros and cons
Abbreviations.
Acetylcholinesterase
Alzheimer’s disease
Amyloid precursor protein
Behavioural and psychological signs and symptoms of dementia
Choline O-acetyltransferase
Disrupted in schizophrenia-1 gene
Diagnostic and Statistical Manual of Mental Disorders
Dysbindin gene
Neuregulin 1 receptor gene
Flinders resistant line
Flinders sensitive line
High-anxiety-related behaviour Wistar rat line
Low-anxiety-related behaviour Wistar rat line
Latent inhibition
Neurofibrillary tangle
Nerve growth factor
N-methyl- d -aspartate
Neuropsychiatric symptoms
Neuregulin 1 gene
Phencyclidine
Platelet-derived growth factor promoter-driven APP
Prepulse inhibition
Research Domain Criteria
Reelin gene
Senescence-accelerated mouse
SAM-prone substrain
Single nucleotide polymorphism
TAR DNA-binding protein 43
Abi-Dargham A (2020) From “bedside” to “bench” and back: A translational approach to studying dopamine dysfunction in schizophrenia. Neurosci Biobehav Rev 110:174–179
Google Scholar
Aghajanian GK, Marek GJ (2000) Serotonin model of schizophrenia: emerging role of glutamate mechanisms. Brain Res Brain Res Rev 31:302–312
Article CAS PubMed Google Scholar
Alonso JR, HS U, Amaral DG (1996) Cholinergic innervation of the primate hippocampal formation: II. Effects of fimbria/fornix transection J Comp Neurol 375:527–551
Amann LC, Gandal MJ, Halene TB et al (2010) Mouse behavioral endophenotypes for schizophrenia. Brain Res Bull 83:147–161
Article PubMed Google Scholar
American Psychiatric Association (2000) Diagnostic and statistical manual of mental health disorders, 4th edn. American Psychiatric Publishing, Washington, DC. https://doi.org/10.1176/appi.books.9780890423349
American Psychiatric Association (2013) Diagnostic and statistical manual of mental disorders, American Psychiatric Publishing, 5th edn, Washington, DC. https://doi.org/10.1176/appi.books.9780890425596
Anderzhanova E, Kirmeier T, Wotjak CT (2017) Animal models in psychiatric research: The RDoC system as a new framework for endophenotype-oriented translational neuroscience. Neurobiol Stress 25:47–56
Article Google Scholar
Andiné P, Widermark N, Axelsson R et al (1999) Characterization of MK-801-induced behavior as a putative rat model of psychosis. J Pharmacol Exp Ther 290:1393–1408
PubMed Google Scholar
Andreasen NC (1995) Symptoms, signs, and diagnosis of schizophrenia. Lancet 346:477–481
Andreasson KI, Savonenko A, Vidensky S et al (2001) Age-dependent cognitive deficits and neuronal apoptosis in cyclooxygenase-2 transgenic mice. J Neurosci 21:8198–8209
Article CAS PubMed PubMed Central Google Scholar
Ashcroft AE (2010) Mass spectrometry and the amyloid problem–how far can we go in the gas phase? J Am Soc Mass Spectrom 21:1087–1096
Avram M, Brandl F, Cabello J et al (2019) Reduced striatal dopamine synthesis capacity in patients with schizophrenia during remission of positive symptoms. Brain 142:1813–1826
Ayhan Y, Abazyan B, Nomura J, Kim R et al (2011) Differential effects of prenatal and postnatal expressions of mutant human DISC1 on neurobehavioral phenotypes in transgenic mice: evidence for neurodevelopmental origin of major psychiatric disorders. Mol Psychiatry 16:293–306
Baker M (2011) Animal models: inside the minds of mice and men. Nature 475:123–128
Banerjee A, Macdonald ML, Borgmann-Winter KE et al (2010) Neuregulin 1-erbB4 pathway in schizophrenia: from genes to an interactome. Brain Res Bull 83:132–139
Barr AM, Fish KN, Markou A et al (2008) Heterozygous reeler mice exhibit alterations in sensorimotor gating but not presynaptic proteins. Eur J Neurosci 27:2568–2574
Basak JM, Holtzman DM (2011) APP-based transgenic models: the PDAPP model. In: De Deyn PP, Van Dam D (eds) Animal models of dementia, 1st edn. Springer Science + Business Media, New York
Bauman MD, Lesh TA, Rowland DJ et al (2019) Preliminary evidence of increased striatal dopamine in a nonhuman primate model of maternal immune activation. Transl Psychiatry 9:135
Article PubMed PubMed Central Google Scholar
Belzung C, Philippot P (2007) Anxiety from a phylogenetic perspective: is there a qualitative difference between human and animal anxiety? Neural Plast 2007:59676
Berlanga ML, Price DL, Phung BS et al (2011) Multiscale imaging characterization of dopamine transporter knockout mice reveals regional alterations in spine density of medium spiny neurons. Brain Res 1390:41–49
Billings LM, Oddo S, Green KN et al (2005) Intraneuronal abeta causes the onset of early Alzheimer’s disease-related cognitive deficits in transgenic mice. Neuron 45:675–688
Bleiholder C, Dupuis NF, Wyttenbach T et al (2011) Ion mobility-mass spectrometry reveals a conformational conversion from random assembly to β-sheet in amyloid fibril formation. Nat Chem 3:172–177
Bons N, Mestre N, Ritchie K et al (1994) Identification of amyloid beta protein in the brain of the small, short-lived lemurian primate microcebus murinus. Neurobiol Aging 15:215–220
Braak H, Braak E, Strothjohann M (1994) Abnormally phosphorylated tau protein related to the formation of neurofibrillary tangles and neuropil threads in the cerebral cortex of sheep and goat. Neurosci Lett 171:1–4
Bray NJ (2008) Gene expression in the etiology of schizophrenia. Schizophr Bull 34:412–418
Brigman JL, Padukiewicz KE, Sutherland ML et al (2006) Executive functions in the heterozygous reeler mouse model of schizophrenia. Behav Neurosci 120:984–998
Brunzell DH, McIntosh JM (2012) Alpha7 nicotinic acetylcholine receptors modulate motivation to self-administer nicotine: implications for smoking and schizophrenia. Neuropsychopharmacology 37:1134–1143
Buccafusco JJ (2008) Methods of behavior analysis in neuroscience. CRC Press/Taylor & Francis Group, Boca Raton
Book Google Scholar
Buka SL, Cannon TD, Torrey EF, Collaborative Study Group on the Perinatal Origins of Severe Psychiatric Disorders et al (2008) Maternal exposure to herpes simplex virus and risk of psychosis among adult offspring. Biol Psychiatry 63:809–815
Büki A, Horvath G, Benedek G et al (2019) Impaired GAD1 expression in schizophrenia-related WISKET rat model with sex-dependent aggressive behavior and motivational deficit. Genes Brain Behav 18:e12507
Article PubMed CAS Google Scholar
Bullock AE, Slobe BS, Vazquez V et al (1997) Inbred mouse strains differ in the regulation of startle and prepulse inhibition of the startle response. Behav Neurosci 111:1353–1360
Bunsey M, Eichenbaum H (1996) Conservation of hippocampal memory function in rats and humans. Nature 379:255–257
Butterfield DA, Poon HF (2005) The senescence-accelerated prone mouse (SAMP8): a model of age-related cognitive decline with relevance to alterations of the gene expression and protein abnormalities in Alzheimer’s disease. Exp Gerontol 40:774–783
Capsoni S, Ugolini G, Comparini A et al (2000) Alzheimer-like neurodegeneration in aged antinerve growth factor transgenic mice. Proc Natl Acad Sci U S A 97:6826–6831
Cardno AG, Marshall EJ, Coid B et al (1999) Heritability estimates for psychotic disorders: the Maudsley twin psychosis series. Arch Gen Psychiatry 56:162–168
Carpenter AP Jr, Pontecorvo MJ, Hefti FF et al (2009) The use of the exploratory IND in the evaluation and development of 18F-PET radiopharmaceuticals for amyloid imaging in the brain: a review of one company’s experience. Q J Nucl Med Mol Imaging 53:387–393
Carpenter WT, Koenig JI (2008) The evolution of drug development in schizophrenia: past issues and future opportunities. Neuropsychopharmacology 33:2061–2079
Castañé A, Theobald DE, Robbins TW (2010) Selective lesions of the dorsomedial striatum impair serial spatial reversal learning in rats. Behav Brain Res 210:74–83
Castellani RJ, Alexiev BA, Phillips D et al (2007) Microscopic investigations in neurodegenerative diseases. In: Méndez-Vilas A, Díaz J (eds) Modern research and educational topics in microscopy. Formatex, Badajoz
Castner SA, al-Tikriti MS, Baldwin RM et al (2000) Behavioral changes and [123I]IBZM equilibrium SPECT measurement of amphetamine-induced dopamine release in rhesus monkeys exposed to subchronic amphetamine. Neuropsychopharmacology 22:4–13
Castner SA, Goldman-Rakic PS (1999) Long-lasting psychotomimetic consequences of repeated low-dose amphetamine exposure in rhesus monkeys. Neuropsychopharmacology 20:10–28
Castner SA, Goldman-Rakic PS (2003) Amphetamine sensitization of hallucinatory-like behaviors is dependent on prefrontal cortex in nonhuman primates. Biol Psychiatry 54:105–110
Caviness VS Jr (1976) Patterns of cell and fiber distribution in the neocortex of the reeler mutant mouse. J Comp Neurol 170:435–447
Chen CJ, Bando K, Ashino H et al (2015) In vivo SPECT imaging of amyloid-β deposition with radioiodinated imidazo[1,2-a]pyridine derivative DRM106 in a mouse model of Alzheimer's disease. J Nucl Med 56:120–126
Cheng Y, Ono M, Kimura H et al (2012) Technetium-99m labeled pyridyl benzofuran derivatives as single photon emission computed tomography imaging probes for β-amyloid plaques in Alzheimer’s brains. J Med Chem 55:2279–2286
Choi SR, Golding G, Zhuang Z et al (2009) Preclinical properties of 18F-AV-45: a PET agent for Abeta plaques in the brain. J Nucl Med 50:1887–1894
Chourbaji S, Zacher C, Sanchis-Segura C et al (2005) Learned helplessness: validity and reliability of depressive-like states in mice. Brain Res Brain Res Protoc 16:70–78
Cistaro A, Alongi P, Caobelli F, Cassalia L (2018) Radiotracers for Amyloid Imaging in Neurodegenerative Disease: State-of-the-Art and Novel Concepts. Curr Med Chem 25:3131–3140
Clapcote SJ, Roder JC (2006) Deletion polymorphism of Disc1 is common to all 129 mouse substrains: implications for gene-targeting studies of brain function. Genetics 173:2407–2410
Cohen BD, Rosenbaum G, Luby ED et al (1962) Comparison of phencyclidine hydrochloride (Sernyl) with other drugs. Simulation of schizophrenic performance with phencyclidine hydrochloride (Sernyl), lysergic acid diethylamide (LSD-25), and amobarbital (Amytal) sodium; II. Symbolic and sequential thinking. Arch Gen Psychiatry 6:395–401
Cohen RM, Rezai-Zadeh K, Weitz TM et al (2013 Apr 10) (2013) A transgenic Alzheimer rat with plaques, tau pathology, behavioral impairment, oligomeric aβ, and frank neuronal loss. J Neurosci 33(15):6245–6256
Colton CA, Wilcock DM, Wink DA et al (2008) The effects of NOS2 gene deletion on mice expressing mutated human AbetaPP. J Alzheimers Dis 15:571–587
Contestabile A (2011) The history of the cholinergic hypothesis. Behav Brain Res 221:334–340
Corcoba A, Steullet P, Duarte JM et al (2015) Glutathione deficit affects the integrity and function of the Fimbria/Fornix and anterior commissure in mice: relevance for Schizophrenia. Int J Neuropsychopharmacol 19:pyv110
Article PubMed PubMed Central CAS Google Scholar
Cork LC, Powers RE, Selkoe DJ et al (1988) Neurofibrillary tangles and senile plaques in aged bears. J Neuropathol Exp Neurol 47:629–641
Crawley J (2000) What’s wrong with my mouse? Behavioral phenotyping of transgenic and knockout mice. Wiley-Liss, Wilmington
Crawley JN, Belknap JK, Collins A et al (1997) Behavioral phenotypes of inbred mouse strains: implications and recommendations for molecular studies. Psychopharmacology 132:107–124
Creese I, Iversen SD (1973) Blockage of amphetamine induced motor stimulation and stereotypy in the adult rat following neonatal treatment with 6-hydroxydopamine. Brain Res 55:369–382
Cui M, Ono M, Kimura H et al (2011) Radioiodinated benzimidazole derivatives as single photon emission computed tomography probes for imaging of β-amyloid plaques in Alzheimer’s disease. Nucl Med Biol 38:313–320
Cummings BJ, Head E, Ruehl W et al (1996) The canine as an animal model of human aging and dementia. Neurobiol Aging 17:259–268
Cummings BJ, Su JH, Cotman CW et al (1993) Beta-amyloid accumulation in aged canine brain: a model of early plaque formation in Alzheimer’s disease. Neurobiol Aging 14:547–560
D’Hooge R, De Deyn PP (2001) Applications of the Morris water maze in the study of learning and memory. Brain Res Brain Res Rev 36:60–90
Davies P, Maloney AJ (1976) Selective loss of central cholinergic neurons in Alzheimer’s disease. Lancet 2:1403
De Deyn PP, Katz IR, Brodathy H et al (2005) Management of agitation, aggression, and psychosis associated with dementia: a pooled analysis including three randomized, placebo-controlled double-blind trials in nursing home residents treated with risperidone. Clin Neurol Neurosurg 107:497–508
De Deyn PP, Van Dam D (2011) General introduction to animal models of human conditions. In: De Deyn PP, Van Dam D (eds) Animal models of dementia, 1st edn. Springer Science + Business Media, New York
Chapter Google Scholar
Deacon R (2011) APP-based transgenic models: the Tg2576 model. In: De Deyn PP, Van Dam D (eds) Animal models of dementia, 1st edn. Springer Science + Business Media, New York
DeBay DR, Reid GA, Pottie IR et al (2017) Targeting butyrylcholinesterase for preclinical single photon emission computed tomography (SPECT) imaging of Alzheimer’s disease. Alzheimers Dement (N Y) 3:166–176
Desbonnet L, Waddington JL, O’Tuathaigh CM (2009) Mutant models for genes associated with schizophrenia. Biochem Soc Trans 37:308–312
Dieckmann M, Freudenberg F, Klein S et al (2007) Disturbed social behavior and motivation in rats selectively bred for deficient sensorimotor gating. Schizophr Res 97:250–253
Doorduin J, de Vries EF, Willemsen AT et al (2009) Neuroinflammation in schizophrenia-related psychosis: a PET study. J Nucl Med 50:1801–1807
Doorduin J, Klein HC, de Jong JR et al (2010) Evaluation of [11C]-DAA1106 for imaging and quantification of neuroinflammation in a rat model of herpes encephalitis. Nucl Med Biol 37:9–15
Drew LJ, Stark KL, Fénelon K et al (2011) Evidence for altered hippocampal function in a mouse model of the human 22q11.2 microdeletion. Mol Cell Neurosci 47:293–305
Dringenberg HC (2000) Alzheimer’s disease: more than a ‘cholinergic disorder’—evidence that cholinergic–monoaminergic interactions contribute to EEG slowing and dementia. Behav Brain Res 115:235–249
Dworkin RH, Opler LA (1992) Simple schizophrenia, negative symptoms, and prefrontal hypodopaminergia. Am J Psychiatry 149:1284–1285
Ebert U, Kirch W (1998) Scopolamine model of dementia: electroencephalogram findings and cognitive performance. Eur J Clin Investig 28:944–949
Article CAS Google Scholar
Eckelman WC (2003) The use of PET and knockout mice in the drug discovery process. Drug Discov Today 8:404–410
Ellenbroek BA, Cools AR (1990) Animal models with construct validity for schizophrenia. Behav Pharmacol 1:469–490
Ellman GL, Courtney KD, Andres J Jr et al (1961) A new and rapid colorimetric determination of acetylcholinesterase activity. Biochem Pharmacol 7:88–95
Erickson CA, Barnes CA (2003) The neurobiology of memory changes in normal aging. Exp Gerontol 38:61–69
Estapé N, Steckler T (2002) Cholinergic blockade impairs performance in operant DNMTP in two inbred strains of mice. Pharmacol Biochem Behav 72:319–334
Fajnerová I, Rodriguez M, Levčík D et al (2014) A virtual reality task based on animal research - spatial learning and memory in patients after the first episode of schizophrenia. Front Behav Neurosci 8:157
Falconer DS (1951) Two new mutants, Trembler and ‘Reeler’, with neurological actions in the house mouse. J Genetics 50:182–201
Farris W, Mansourian S, Chang Y et al (2003) Insulin-degrading enzyme regulates the levels of insulin, amyloid beta-protein, and the beta-amyloid precursor protein intracellular domain in vivo. Proc Natl Acad Sci U S A 100:4162–4167
Featherstone RE, Kapur S, Fletcher PJ (2007) The amphetamine-induced sensitized state as a model of schizophrenia. Prog Neuro-Psychopharmacol Biol Psychiatry 31:1556–1571
Featherstone RE, Rizos Z, Kapur S et al (2008) A sensitizing regimen of amphetamine that disrupts attentional set-shifting does not disrupt working or long-term memory. Behav Brain Res 189:170–179
Femminella GD, Thayanandan T, Calsolaro V et al (2018) Imaging and Molecular Mechanisms of Alzheimer's Disease: A Review. Int J Mol Sci 19. pii: E3702
Flood DG, Zuvich E, Marino MJ et al (2011) Prepulse inhibition of the startle reflex and response to antipsychotic treatments in two outbred mouse strains in comparison to the inbred DBA/2 mouse. Psychopharmacology 215:441–454
Floresco SB, Zhang Y, Enomoto T (2009) Neural circuits subserving behavioral flexibility and their relevance to schizophrenia. Behav Brain Res 204:396–409
Fone KC, Porkess MV (2008) Behavioural and neurochemical effects of post-weaning social isolation in rodents-relevance to developmental neuropsychiatric disorders. Neurosci Biobehav Rev 32:1087–1102
Fonnum F (1975) A rapid radiochemical method for the determination of choline acetyltransferase. J Neurochem 24:407–409
Frautschy SA, Yang F, Calderón L et al (1996) Rodent models of Alzheimer’s disease: rat A beta infusion approaches to amyloid deposits. Neurobiol Aging 17:311–321
Freichel C, Neumann M, Ballard T et al (2007) Age-dependent cognitive decline and amygdala pathology in alpha-synuclein transgenic mice. Neurobiol Aging 28:1421–1435
Freyberg Z, Ferrando SJ, Javitch JA (2010) Roles of the Akt/GSK-3 and Wnt signaling pathways in schizophrenia and antipsychotic drug action. Am J Psychiatry 167:388–396
Fuchigami T, Yamashita Y2, Haratake M et al (2014) Synthesis and evaluation of ethyleneoxylated and allyloxylated chalcone derivatives for imaging of amyloid β plaques by SPECT. Bioorg Med Chem 22:2622–2628
Fuster JM (1980) The prefrontal cortex. Anatomy, physiology, and neuropsychology of the frontal lobe. Raven Press, New York
Games D, Adams D, Alessandrini R et al (1995) Alzheimer-type neuropathology in transgenic mice overexpressing V717F beta-amyloid precursor protein. Nature 373:523–527
Gayle DA, Beloosesky R, Desai M et al (2004) Maternal LPS induces cytokines in the amniotic fluid and corticotropin releasing hormone in the fetal rat brain. Am J Physiol Regul Integr Comp Physiol 286:R1024–R1029
Gearing M, Rebeck GW, Hyman BT et al (1994) Neuropathology and apolipoprotein E profile of aged chimpanzees: implications for Alzheimer’s disease. Proc Natl Acad Sci U S A 91:9382–9386
Gearing M, Tigges J, Mori H et al (1997) β-amyloid (Aβ) deposition in the brains of aged orangutans. Neurobiol Aging 18:139–146
Gejman PV, Sanders AR, Kendler KS (2011) Genetics of schizophrenia: new findings and challenges. Annu Rev Genomics Hum Genet 12:121–144
Gerlai R (2010) Zebrafish antipredatory responses: a future for translational research? Behav Brain Res 207:223–231
Geula C, Nagykery N, Wu CK (2002) Amyloid-beta deposits in the cerebral cortex of the aged common marmoset (Callithrix jacchus): incidence and chemical composition. Acta Neuropathol 103:48–58
Girard SD, Baranger K, Gauthier C et al (2013) Evidence for early cognitive impairment related to frontal cortex in the 5XFAD mouse model of Alzheimer's disease. J Alzheimers Dis 33:781–796
Glahn DC, Thompson PM, Blangero J (2007) Neuroimaging endophenotypes: strategies for finding genes influencing brain structure and function. Hum Brain Mapp 28:488–501
Glenn MJ, Nesbitt C, Mumby DG (2003) Perirhinal cortex lesions produce variable patterns of retrograde amnesia in rats. Behav Brain Res 141:183–193
Glowinski J, Axelrod J, Iversen LL (1966) Regional studies of catecholamines in the rat brain. IV. Effects of drugs on the disposition and metabolism of H3-norepinephrine and H3-dopamine. J Pharmacol Exp Ther 153:30–41
CAS PubMed Google Scholar
Glowinski J, Iversen L (1966a) Regional studies of catecholamines in the rat brain. 3. Subcellular distribution of endogenous and exogenous catecholamines in various brain regions. Biochem Pharmacol 15:977–987
Glowinski J, Iversen LL (1966b) Regional studies of catecholamines in the rat brain. I. The disposition of [3H]norepinephrine, [3H]dopamine and [3H]dopa in various regions of the brain. J Neurochem 13:655–669
Gogos A, Bogeski M, van den Buuse M (2008) Role of serotonin-1A receptors in the action of antipsychotic drugs: comparison of prepulse inhibition studies in mice and rats and relevance for human pharmacology. Behav Pharmacol 19:548–561
Gong Y, Chang L, Viola KL et al (2003) Alzheimer’s disease-affected brain: presence of oligomeric A beta ligands (ADDLs) suggests a molecular basis for reversible memory loss. Proc Natl Acad Sci U S A 100:10417–10422
Gosling SD (2001) From mice to men: what can we learn about personality from animal research? Psychol Bull 127:45–86
Gottesman II, Gould TD (2003) The endophenotype concept in psychiatry: etymology and strategic intentions. Am J Psychiatry 160:636–645
Götz J, Schild A, Hoerndli F et al (2004) Amyloid-induced neurofibrillary tangle formation in Alzheimer’s disease: insight from transgenic mouse and tissue-culture models. Int J Dev Neurosci 22:453–465
Gould TD, Gottesman II (2006) Psychiatric endophenotypes and the development of valid animal models. Genes Brain Behav 5:113–119
Gray JA, McNaughton N (1983) Comparison between the behavioural effects of septal and hippocampal lesions: a review. Neurosci Biobehav Rev 7:119–188
Grayson DR, Chen Y, Costa E et al (2006) The human reelin gene: transcription factors (+), repressors (−) and the methylation switch (+/−) in schizophrenia. Pharmacol Ther 111:272–286
Gsell W, Jungkunz G, Riederer P (2004) Functional neurochemistry of Alzheimer’s disease. Curr Pharm Des 10:265–293
Guidotti A, Auta J, Davis JM et al (2005) GABAergic dysfunction in schizophrenia: new treatment strategies on the horizon. Psychopharmacology 180:191–205
Gulchina Y, Xu SJ, Snyder MA et al (2017) Epigenetic mechanisms underlying NMDA receptor hypofunction in the prefrontal cortex of juvenile animals in the MAM model for schizophrenia. J Neurochem 143:320–333
Gunn-Moore DA, McVee J, Bradshaw JM et al (2006) Ageing changes in cat brains demonstrated by beta-amyloid and AT8-immunoreactive phosphorylated tau deposits. J Feline Med Surg 8:234–242
Hardy J, Selkoe DJ (2002) The amyloid hypothesis of Alzheimer’s disease: progress and problems on the road to therapeutics. Science 297:353–356
Harkany T, O’Mahony S, Kelly JP et al (1998) Beta-amyloid(Phe(SO3H)24)25-35 in rat nucleus basalis induces behavioral dysfunctions, impairs learning and memory and disrupts cortical cholinergic innervation. Behav Brain Res 90:133–145
Harkany T, Penke B, Luiten PG (2000) beta-Amyloid excitotoxicity in rat magnocellular nucleus basalis. Effect of cortical deafferentation on cerebral blood flow regulation and implications for Alzheimer’s disease. Ann N Y Acad Sci 903:374–386
Harrison P, Law A (2006) Neuregulin 1 and schizophrenia: genetics, gene expression, and neurobiology. Biol Psychiatry 60:132–140
Hattori S, Murotani T, Matsuzaki S et al (2008) Behavioral abnormalities and dopamine reductions in sdy mutant mice with a deletion in Dtnbp1, a susceptibility gene for schizophrenia. Biochem Biophys Res Commun 373:298–302
Hauss-Wegrzyniak B, Dobrzanski P, Stoehr JD et al (1998) Chronic neuroinflammation in rats reproduces components of the neurobiology of Alzheimer’s disease. Brain Res 780:294–303
He Y, Yao Z, Gu Y et al (1992) Nerve growth factor promotes collateral sprouting of cholinergic fibers in the septohippocampal cholinergic system of aged rats with fimbria transection. Brain Res 586:27–35
Head E, Moffat K, Das P et al (2005) β-amyloid deposition and tau phosphorylation in clinically characterized aged cats. Neurobiol Aging 26:749–763
Hendley ED, Welch BL (1975) Electroconvulsive shock: sustained decrease in norepinephrine uptake affinity in a reserpine model of depression. Life Sci 16:45–54
Herth MM, Petersen IN, Hansen HD et al (2016) Synthesis and evaluation of (18)F-labeled 5-HT2A receptor agonists as PET ligands. Nucl Med Biol 43:455–462
Hohmann CF, Beard NA, Kari-Kari P et al (2012) Effects of brief stress exposure during early postnatal development in Balb/CByJ mice: II. Altered cortical morphology. Dev Psychobiol 54:723–735
Horti AG, Gao Y, Kuwabara H, Dannals RF (2010) Development of radioligands with optimized imaging properties for quantification of nicotinic acetylcholine receptors by positron emission tomography. Life Sci 86:575–584
Horvath G, Petrovszki Z, Kekesi G et al (2016) Electrophysiological alterations in a complex rat model of schizophrenia. Behav Brain Res 307:65–72
Hsiao K, Chapman P, Nilsen S et al (1996) Correlative memory deficits, Abeta elevation, and amyloid plaques in transgenic mice. Science 274:99–102
Huang Y, Zheng MQ, Gerdes JM et al (2010) Development of effective PET and SPECT imaging agents for the serotonin transporter: has a twenty-year journey reached its destination? Curr Top Med Chem 10:1499–1526
Huber G, März W, Martin JR et al (2000) Characterization of transgenic mice expressing apolipoprotein E4(C112R) and apolipoprotein E4(L28P; C112R). Neuroscience 101:211–218
Iliadi KG (2009) The genetic basis of emotional behavior: has the time come for a Drosophila model? J Neurogenet 23:136–146
Insel TR (2007) From animal model to model animals. Biol Psychiatry 62:1337–1339
Ishrat T, Parveen K, Khan MM et al (2009) Selenium prevents cognitive decline and oxidative damage in rat model of streptozotocin-induced experimental dementia of Alzheimer’s type. Brain Res 1281:117–127
Iversen LL, Glowinski J (1966) Regional studies of catecholamines in the rat brain. II Rate of turnover of catecholamines in various brain regions J Neurochem 13:671–682
Iwata N, Tsubuki S, Takaki Y et al (2001) Metabolic regulation of brain Abeta by neprilysin. Science 292:1550–1552
Jaaro-Peled H (2009) Gene models of schizophrenia: DISC1 mouse models. Prog Brain Res 179:75–86
Jaehne EJ, Ramshaw H, Xu X et al (2015) In-vivo administration of clozapine affects behaviour but does not reverse dendritic spine deficits in the 14-3-3ζ KO mouse model of schizophrenia-like disorders. Pharmacol Biochem Behav 138:1–8
Javitt DC, Zukin SR (1991) Recent advances in the phencyclidine model of schizophrenia. Am J Psychiatry 148:1301–1308
Jawhar S, Trawicka A, Jenneckens C et al (2012) Motor deficits, neuron loss, and reduced anxiety coinciding with axonal degeneration and intraneuronal Aβ aggregation in the 5XFAD mouse model of Alzheimer's disease. Neurobiol Aging 33:196.e29-40
Jaworski T, Dewachter I, Seymour CM et al (2010) Alzheimer’s disease: old problem, new views from transgenic and viral models. Biochim Biophys Acta 1802:808–818
Jentsch JD, Roth RH (1999) The neuropsychopharmacology of phencyclidine: from NMDA receptor hypofunction to the dopamine hypothesis of schizophrenia. Neuropsychopharmacology 20:201–225
Johnson KA, Minoshima S, Bohnen NI et al (2013) Appropriate use criteria for amyloid PET: a report of the Amyloid Imaging Task Force, the Society of Nuclear Medicine and Molecular Imaging, and the Alzheimer’s Association. J Nucl Med 54:476–490
Jones CA, Watson DJ, Fone KC (2011) Animal models of schizophrenia. Br J Pharmacol 164:1162–1194
Kaimal V, McConville P (2009) Importance of preclinical imaging in drug discovery. Charles River. https://pdfs.semanticscholar.org/f049/5930b61a7c117704f1467a98aa1e75dd1d3e.pdf . Accessed 23 July 2019
Kambeitz J, Abi-Dargham A, Kapur S, Howes OD (2014) Alterations in cortical and extrastriatal subcortical dopamine function in schizophrenia: systematic review and meta-analysis of imaging studies. Br J Psychiatry 204:420–429
Kapur S, Remington G (1996) Serotonin-dopamine interaction and its relevance to schizophrenia. Am J Psychiatry 153:466–476
Kekesi G, Petrovszki Z, Benedek G, Horvath G (2015) Sex-specific alterations in behavioral and cognitive functions in a "three hit" animal model of schizophrenia. Behav Brain Res 284:85–93
Kilbourn MR, Domino EF (2011) Increased in vivo [11C]raclopride binding to brain dopamine receptors in amphetamine-treated rats. Eur J Pharmacol 654:254–257
Kim S, Jung WH2, Howes OD et al (2018) Frontostriatal functional connectivity and striatal dopamine synthesis capacity in schizophrenia in terms of antipsychotic responsiveness: an [18F]DOPA PET and fMRI study. Psychol Med 21:1–10
Kimura N, Tanemura K, Nakamura S et al (2003) Age-related changes of Alzheimer’s disease-associated proteins in cynomolgus monkey brains. Biochem Biophys Res Commun 310:303–311
Kinney GG, Wilkinson LO, Saywell KL et al (1999) Rat strain differences in the ability to disrupt sensorimotor gating are limited to the dopaminergic system, specific to prepulse inhibition, and unrelated to changes in startle amplitude or nucleus accumbens dopamine receptor sensitivity. J Neurosci 19:5644–5653
Kirino S, Suzuki T, Takeuchi H (2017) Representativeness of clinical PET study participants with schizophrenia: A systematic review. J Psychiatr Res 88:72–79
Kiritsis C, Mavroidi B, Shegani A et al (2017) 2-(4'-Aminophenyl)benzothiazole Labeled with 99mTc-Cyclopentadienyl for Imaging β-Amyloid Plaques. ACS Med Chem Lett 8:1089–1092
Klein S, Koch M, Schwabe K (2008) Neuroanatomical changes in the adult rat brain after neonatal lesion of the medial prefrontal cortex. Exp Neurol 209:199–212
Kleinberger G, Brendel M, Mracsko E et al (2017) The FTD-like syndrome causing TREM2 T66M mutation impairs microglia function, brain perfusion, and glucose metabolism. EMBO J 36:1837–1853
Klunk WE, Engler H, Nordberg A et al (2004) Imaging brain amyloid in Alzheimer’s disease with Pittsburgh Compound-B. Ann Neurol 55:306–319
Klunk WE, Lopresti BJ, Ikonomovic MD et al (2005) Binding of the positron emission tomography tracer Pittsburgh compound-B reflects the amount of amyloid-beta in Alzheimer’s disease brain but not in transgenic mouse brain. J Neurosci 25:10598–10606
Koike H, Arguello PA, Kvajo M et al (2006) Disc1 is mutated in the 129S6/SvEv strain and modulates working memory in mice. Proc Natl Acad Sci U S A 103:3693–3697
Kokkinidis L, Anisman H (1981) Amphetamine psychosis and schizophrenia: a dual model. Neurosci Biobehav Rev 5:449–461
Koric L, Guedj E, Habert MO et al (2016) Molecular imaging in the diagnosis of Alzheimer's disease and related disorders. Rev Neurol (Paris) 172:725–734
Krystal JH, Karper LP, Seibyl JP et al (1994) Subanesthetic effects of the noncompetitive NMDA antagonist, ketamine, in humans. Psychotomimetic, perceptual, cognitive, and neuroendocrine responses. Arch Gen Psychiatry 51:199–214
Kuntner C, Kesner AL, Bauer M et al (2009) Limitations of small animal PET imaging with [18F]FDDNP and FDG for quantitative studies in a transgenic mouse model of Alzheimer’s disease. Mol Imaging Biol 11:236–240
Lacor PN, Buniel MC, Chang L et al (2004) Synaptic targeting by Alzheimer’s-related amyloid beta oligomers. J Neurosci 24:10191–10200
Laczó J, Andel R, Vyhnalek M et al (2010) Human analogue of the morris water maze for testing subjects at risk of Alzheimer's disease. Neurodegener Dis 7:148–152
Lane MA (2000) Nonhuman primate models in biogerontology. Exp Gerontol 35:533–541
Laviola G, Ognibene E, Romano E et al (2009) Gene-environment interaction during early development in the heterozygous reeler mouse: clues for modelling of major neurobehavioral syndromes. Neurosci Biobehav Rev 33:560–572
Lawlor PA, Young D (2011) Aβ infusion and related models of Alzheimer dementia. In: De Deyn PP, Van Dam D (eds) Animal models of dementia, 1st edn. Springer Science + Business Media, New York
Lazar NL, Rajakumar N, Cain DP (2008) Injections of NGF into neonatal frontal cortex decrease social interaction as adults: a rat model of schizophrenia. Schizophr Bull 34:127–136
Leboyer M, Bellivier F, Nosten-Bertrand M et al (1998) Psychiatric genetics: search for phenotypes. Trends Neurosci 21:102–105
Lemere CA, Beierschmitt A, Iglesias M et al (2004) Alzheimer’s disease abeta vaccine reduces central nervous system abeta levels in a non-human primate, the Caribbean vervet. Am J Pathol 165:283–297
Lemere CA, Oh J, Stanish HA et al (2008) Cerebral amyloid-beta protein accumulation with aging in cotton-top tamarins: a model of early Alzheimer’s disease? Rejuvenation Res 11:321–332
Lescaudron L, Stein DG (1999) Differences in memory impairment and response to GM1 ganglioside treatment following electrolytic or ibotenic acid lesions of the nucleus basalis magnocellularis. Restor Neurol Neurosci 15:25–37
Leung C, Jia Z (2016) Mouse Genetic Models of Human Brain Disorders. Front Genet 7:40
Levin ED, Rose JE, McGurk SR et al (1990) Characterization of the cognitive effects of combined muscarinic and nicotinic blockade. Behav Neural Biol 53:103–112
Lewis DA, Levitt P (2002) Schizophrenia as a disorder of neurodevelopment. Annu Rev Neurosci 25:409–432
Li Q, Cheung C, Wei R et al (2009) Prenatal immune challenge is an environmental risk factor for brain and behavior change relevant to schizophrenia: evidence from MRI in a mouse model. PLoS One 4:e6354
Liebsch G, Linthorst AC, Neumann ID et al (1998) Behavioral, physiological, and neuroendocrine stress responses and differential sensitivity to diazepam in two Wistar rat lines selectively bred for high- and low-anxiety-related behavior. Neuropsychopharmacology 19:381–396
Lipska BK (2004) Using animal models to test a neurodevelopmental hypothesis of schizophrenia. J Psychiatry Neurosci 29:282–286
PubMed PubMed Central Google Scholar
Lipska BK, Weinberger DR (2000) To model a psychiatric disorder in animals: schizophrenia as a reality test. Neuropsychopharmacology 23:223–239
Liu L, Duff K (2008) A technique for serial collection of cerebrospinal fluid from the cisterna magna in mouse. J Vis Exp 21:e960
Lodge DJ, Grace AA (2008) Hippocampal dysfunction and disruption of dopamine system regulation in an animal model of schizophrenia. Neurotox Res 14:97–104
Lubow RE, Gewirtz JC (1995) Latent inhibition in humans: data, theory, and implications for schizophrenia. Psychol Bull 117:87–103
Luo F, Rustay NR, Ebert U et al (2012) Characterization of 7- and 19-month-old Tg2576 mice using multimodal in vivo imaging: limitations as a translatable model of Alzheimer’s disease. Neurobiol Aging 33:933–944
Lyketsos CG, Carrillo MC, Ryan JM et al (2011) Neuropsychiatric symptoms in Alzheimer's disease. Alzheimers Dement 7:532–539
Maass A, Landau S, Baker SL et al (2017) Comparison of multiple tau-PET measures as biomarkers in aging and Alzheimer's disease. NeuroImage 157:448–463
MacLean PD (1970) The Triune Brain, Emotion and Scientific Bias. In: Schmitt FO (Ed.) The Neurosciences: Second Study Program. New York: Rockefeller University Press, 336–349
Maeda J, Ji B, Irie T et al (2007) Longitudinal, quantitative assessment of amyloid, neuroinflammation, and anti-amyloid treatment in a living mouse model of Alzheimer’s disease enabled by positron emission tomography. J Neurosci 27:10957–10968
Marcotte ER, Pearson DM, Srivastava LK (2001) Animal models of schizophrenia: a critical review. J Psychiatry Neurosci 26:395–410
CAS PubMed PubMed Central Google Scholar
Markham JA, Taylor AR, Taylor SB et al (2010) Characterization of the cognitive impairments induced by prenatal exposure to stress in the rat. Front Behav Neurosci 4:173
Marona-Lewicka D, Nichols CD, Nichols DE (2011) An animal model of schizophrenia based on chronic LSD administration: old idea, new results. Neuropharmacology 61:503–512
Martínez-Téllez RI, Hernández-Torres E, Gamboa C et al (2009) Prenatal stress alters spine density and dendritic length of nucleus accumbens and hippocampus neurons in rat offspring. Synapse 63:794–804
Martins AF, Morfin JF, Kubíčková A et al (2013) PiB-Conjugated, Metal-Based Imaging Probes: Multimodal Approaches for the Visualization of β-Amyloid Plaques. ACS Med Chem Lett 4:436–440
Maximino C, van der Staay FJ (2019) Behavioral models in psychopathology: epsitemic and semantic considerations. Behav Brain Funct 15:1
McGeer EG, McGeer PL (2003) Inflammatory processes in Alzheimer’s disease. Prog Neuro-Psychopharmacol Biol Psychiatry 27:741–749
McGrath J, Saha S, Chant D et al (2008) Schizophrenia: a concise overview of incidence, prevalence, and mortality. Epidemiol Rev 30:67–76
McKinney WT Jr, Bunney WE Jr (1969) Animal model of depression. I. Review of evidence: implications for research. Arch Gen Psychiatry 21:240–248
Mei L, Xiong W (2008) Neuregulin 1 in neural development, synaptic plasticity and schizophrenia. Nat Rev Neurosci 9:437–452
Mhatre SD, Paddock BE, Saunders AJ, Marenda DR (2013) Invertebrate models of Alzheimer's disease. J Alzheimers Dis 33:3–16
Migliore L, Fontana I, Colognato R et al (2005) Searching for the role and the most suitable biomarkers of oxidative stress in Alzheimer’s disease and in other neurodegenerative diseases. Neurobiol Aging 26:587–595
Milak MS, Severance AJ, Ogden RT et al (2008) Modeling considerations for 11C-CUMI-101, an agonist radiotracer for imaging serotonin 1A receptor in vivo with PET. J Nucl Med 49:587–596
Mitchell KJ, Huang ZJ, Moghaddam B et al (2011) Following the genes: a framework for animal modelling of psychiatric disorders. BMC Biol 9:76
Molavipordanjani S, Emami S, Mardanshahi A et al (2019) Novel 99m Tc-2-arylimidazo[2,1-b]benzothiazole derivatives as SPECT imaging agents for amyloid-β plaques. Eur J Med Chem 175:149–161
Moore H (2010) The role of rodent models in the discovery of new treatments for schizophrenia: updating our strategy. Schizophr Bull 36:1066–1072
Moran P, Stokes J, Marr J et al (2016) Gene × Environment Interactions in Schizophrenia: Evidence from Genetic Mouse Models. Neural Plast 2173748
Moran PM (1993) Differential effects of scopolamine and mecamylamine on working and reference memory in the rat. Pharmacol Biochem Behav 45:533–538
Moreno JL, Holloway T, Umali A et al (2013) Persistent effects of chronic clozapine on the cellular and behavioral responses to LSD in mice. Psychopharmacology 225:217–226
Muir WJ, Pickard BS, Blackwood DH (2008) Disrupted-in-schizophrenia-1. Curr Psychiatry Rep 10:140–147
Mulder J, Harkany T, Czollner K et al (2005) Galantamine-induced behavioral recovery after sublethal excitotoxic lesions to the rat medial septum. Behav Brain Res 163:33–41
Nag S, Yee BK, Tang F (1999) Chronic intracerebroventricular infusion of beta-amyloid (1-40) results in a selective loss of neuropeptides in addition to a reduction in choline acetyltransferase activity in the cortical mantle and hippocampus in the rat. Ann N Y Acad Sci 897:420–422
Nakamura H, Hishinuma T, Tomioka Y et al (1997) Effects of haloperidol and cocaine pretreatments on brain distribution and kinetics of [11C]methamphetamine in methamphetamine sensitized dog: application of PET to drug pharmacokinetic study. Nucl Med Biol 24:165–169
Nakamura S, Murayama N, Noshita T et al (2001) Progressive brain dysfunction following intracerebroventricular infusion of beta(1-42)-amyloid peptide. Brain Res 912:128–136
Nakao A, Miyazaki N2, Ohira K et al (2017) Immature morphological properties in subcellular-scale structures in the dentate gyrus of Schnurri-2 knockout mice: a model for schizophrenia and intellectual disability. Mol Brain 10:60
Nestler EJ, Hyman SE (2010) Animal models of neuropsychiatric disorders. Nat Neurosci 13:1161–1169
Neuman KM, Molina-Campos E, Musial TF et al (2015) Evidence for Alzheimer's disease-linked synapse loss and compensation in mouse and human hippocampal CA1 pyramidal neurons. Brain Struct Funct 220:3143–3165
Nordberg A, Nilsson-Håkansson L, Adem A et al (1989) Multiple actions of THA on cholinergic neurotransmission in Alzheimer brains. Prog Clin Biol Res 317:1169–1178
Nullmeier S, Panther P, Frotscher M et al (2014) Alterations in the hippocampal and striatal catecholaminergic fiber densities of heterozygous reeler mice. Neuroscience 275:404–419
O’Tuathaigh CM, Kirby BP, Moran PM et al (2010) Mutant mouse models: genotype-phenotype relationships to negative symptoms in schizophrenia. Schizophr Bull 36:271–288
Oakley H, Cole SL, Logan S et al (2006) Intraneuronal beta-amyloid aggregates, neurodegeneration, and neuron loss in transgenic mice with five familial Alzheimer's disease mutations: potential factors in amyloid plaque formation. J Neurosci 26:10129–10140
Oddo S, Caccamo A, Kitazawa M et al (2003) Amyloid deposition precedes tangle formation in a triple transgenic model of Alzheimer’s disease. Neurobiol Aging 24:1063–1070
Okamura N, Harada R, Furumoto S et al (2014) Tau PET imaging in Alzheimer's disease. Curr Neurol Neurosci Rep 14:500
Okubo Y, Suhara T, Suzuki K et al (1997) Decreased prefrontal dopamine D1 receptors in schizophrenia revealed by PET. Nature 385:634–636
Olariu A, Yamada K, Mamiya T et al (2002) Memory impairment induced by chronic intracerebroventricular infusion of beta-amyloid (1-40) involves downregulation of protein kinase C. Brain Res 957:278–286
Olney JW, Farber NB (1995) Glutamate receptor dysfunction and schizophrenia. Arch Gen Psychiatry 52:998–1007
Ono M, Watanabe H, Kitada A et al (2016) Highly Selective Tau-SPECT Imaging Probes for Detection of Neurofibrillary Tangles in Alzheimer's Disease. Sci Rep 6:34197
Ono M, Cheng Y, Kimura H et al (2013) Development of novel 123I-labeled pyridyl benzofuran derivatives for SPECT imaging of β-amyloid plaques in Alzheimer's disease. PLoS One 8:e74104
Opazo C, Luza S, Villemagne VL et al (2006) Radioiodinated clioquinol as a biomarker for beta-amyloid: Zn complexes in Alzheimer’s disease. Aging Cell 5:69–79
Overstreet DH, Double K, Schiller GD (1989) Antidepressant effects of rolipram in a genetic animal model of depression: cholinergic supersensitivity and weight gain. Pharmacol Biochem Behav 34:691–696
Owens EM, Bachman P, Glahn DC, Bearden CE (2016) Electrophysiological Endophenotypes for Schizophrenia. Harv Rev Psychiatry 24:129–147
Palmer AA, Dulawa SC, Mottiwala AA et al (2000) Prepulse startle deficit in the Brown Norway rat: a potential genetic model. Behav Neurosci 114:374–388
Patel NH, Vyas NS, Puri BK et al (2010) Positron emission tomography in schizophrenia: a new perspective. J Nucl Med 51:511–520
Paylor R, Crawley JN (1997) Inbred strain differences in prepulse inhibition of the mouse startle response. Psychopharmacology 132:169–180
Paylor R, Lindsay E (2006) Mouse models of 22q11 deletion syndrome. Biol Psychiatry 59:1172–1179
Peilin J, Guangchun H, Junfei Z et al (2017) SZGR 2.0: a one-stop shop of schizophrenia candidate genes. Nucleic Acids Res 45(Database issue):D915–D924
Pentkowski NS, Berkowitz LE, Thompson SM et al (2018) Anxiety-like behavior as an early endophenotype in the TgF344-AD rat model of Alzheimer's disease. Neurobiol Aging 61:169–176
Pepeu G, Giovannini MG (2007) Changes in acetylcholine extracellular levels during cognitive processes. In: Westerink BH, Cremers TI (eds) Handbook of microdialysis. Methods, applications and perspectives. Elsevier, Amsterdam
Pepeu G, Rosi MC (2011) Validation of animal models of dementia: neurochemical aspects. In: De Deyn PP, Van Dam D (eds) Animal models of dementia, 1st edn. Springer Science + Business Media, New York
Petersen IN, Villadsen J, Hansen HD et al (2016) Convergent 18F-labeling and evaluation of N-benzyl-phenethylamines as 5-HT2A receptor PET ligands. Bioorg Med Chem 24:5353–5356
Pietropaolo S, Crusio WE (2009) Strain-dependent changes in acoustic startle response and its plasticity across adolescence in mice. Behav Genet 39:623–631
Platt TL, Reeves VL, Murphy MP (2013) Transgenic models of Alzheimer's disease: better utilization of existing models through viral transgenesis. Biochim Biophys Acta 1832:1437–1448
Podhorna J, Didriksen M (2004) The heterozygous reeler mouse: behavioural phenotype. Behav Brain Res 153:43–54
Podlisny MB, Tolan DR, Selkoe DJ (1991) Homology of the amyloid beta protein precursor in monkey and human supports a primate model for beta amyloidosis in Alzheimer’s disease. Am J Pathol 138:1423–1435
Price JC, Klunk WE, Lopresti BJ et al (2005) Kinetic modeling of amyloid binding in humans using PET imaging and Pittsburgh Compound-B. J Cereb Blood Flow Metab 25:1528–1547
Puhl MD, Mintzopoulos D2, Jensen JE et al (2015) In vivo magnetic resonance studies reveal neuroanatomical and neurochemical abnormalities in the serine racemase knockout mouse model of schizophrenia. Neurobiol Dis 73:269–274
Rabinovici GD, Jagust WJ (2009) Amyloid imaging in aging and dementia: testing the amyloid hypothesis in vivo. Behav Neurol 21:117–128
Raedler TJ, Bymaster FP, Tandon R et al (2007) Towards a muscarinic hypothesis of schizophrenia. Mol Psychiatry 12:232–246
Rankin CA, Gamblin TC (2008) Assessing the toxicity of tau aggregation. J Alzheimers Dis 14:411–416
Reardon S (2019) Depression researchers rethink popular mouse swim tests Nature 571: 456–457. https://www.nature.com/articles/d41586-019-02133-?utm_source=fbk_nnc&utm_medium=social&utm_campaign=naturenews&sf216017303=1&fbclid=IwAR0gq-hnRrXCldfHPZdoN-gCnfUY2IfbzqzvHJi5v8WGdBdNAqGp7WSA__E . Accessed 23 July 2019
Reisberg B, Borenstein J, Salob SP et al (1987) Behavioral symptoms in Alzheimer’s disease: phenomenology and treatment. J Clin Psychiatry 48:9–15
Reith J, Cumming P, Gjedde A (1998) Enhanced [3H]DOPA and [3H]dopamine turnover in striatum and frontal cortex in vivo linked to glutamate receptor antagonism. J Neurochem 70:1979–1985
Renã AS, Butterfield DA (2011) Spontaneous vertebrate models of Alzheimer dementia: selectively bred strains (SAM strains). In: De Deyn PP, Van Dam D (eds) Animal models of dementia, 1st edn. Springer Science + Business Media, New York
Ribé EM, Pérez M, Puig B et al (2005) Accelerated amyloid deposition, neurofibrillary degeneration and neuronal loss in double mutant APP/tau transgenic mice. Neurobiol Dis 20:814–822
Riekkinen P Jr, Sirviö J, Aaltonen M et al (1990) Effects of concurrent manipulations of nicotinic and muscarinic receptors on spatial and passive avoidance learning. Pharmacol Biochem Behav 37:405–410
Roertgen KE, Parisi JE, Clark HB et al (1996) A beta-associated cerebral angiopathy and senile plaques with neurofibrillary tangles and cerebral hemorrhage in an aged wolverine (Gulo gulo). Neurobiol Aging 17:243–247
Rofina JE, van Ederen AM, Toussaint MJ et al (2006) Cognitive disturbances in old dogs suffering from the canine counterpart of Alzheimer’s disease. Brain Res 1069:216–226
Rosen AM, Spellman T, Gordon JA (2015) Electrophysiological endophenotypes in rodent models of schizophrenia and psychosis. Biol Psychiatry 77:1041–1049
Rosen RF, Walker LC, Levine H 3rd (2011) PIB binding in aged primate brain: enrichment of high-affinity sites in humans with Alzheimer’s disease. Neurobiol Aging 32:223–234
Sagnou M, Mavroidi B, Shegani A et al (2019) Remarkable Brain Penetration of Cyclopentadienyl M(CO) 3 + (M = 99m Tc, Re) Derivatives of Benzothiazole and Benzimidazole Paves the Way for Their Application as Diagnostic, with Single-Photon-Emission Computed Tomography (SPECT), and Therapeutic Agents for Alzheimer's Disease. J Med Chem 62:2638–2650
Sakoğlu U, Upadhyay J, Chin CL et al (2011) Paradigm shift in translational neuroimaging of CNS disorders. Biochem Pharmacol 81:1374–1387
Sanchis-Segura C, Spanagel R, Henn FA et al (2005) Reduced sensitivity to sucrose in rats bred for helplessness: a study using the matching law. Behav Pharmacol 16:267–270
Sani S, Traul D, Klink A et al (2003) Distribution, progression and chemical composition of cortical amyloid-β deposits in aged rhesus monkeys: similarities to the human. Acta Neuropathol 105:145–156
Santarelli L, Gobbi G, Debs PC et al (2001) Genetic and pharmacological disruption of neurokinin 1 receptor function decreases anxiety-related behaviors and increases serotonergic function. Proc Natl Acad Sci U S A 98:1912–1917
Schmitt A, Turck CW, Pilz PK et al (2013) Proteomic similarities between heterozygous reeler mice and schizophrenia. Biol Psychiatry 74:e5–e10
Seeman P (1987) Dopamine receptors and the dopamine hypothesis of schizophrenia. Synapse 1:133–152
Selkoe DJ (2000) Toward a comprehensive theory for Alzheimer’s disease. Hypothesis: Alzheimer’s disease is caused by the cerebral accumulation and cytotoxicity of amyloid beta-protein. Ann N Y Acad Sci 924:17–25
Selkoe DJ (2001) Alzheimer’s disease: genes, proteins, and therapy. Physiol Rev 81:741–766
Selkoe DJ (2008) Soluble oligomers of the amyloid beta-protein impair synaptic plasticity and behavior. Behav Brain Res 192:106–113
Sherman KA, Friedman E (1990) Pre- and post-synaptic cholinergic dysfunction in aged rodent brain regions: new findings and an interpretive review. Int J Dev Neurosci 8:689–708
Shin J, Kepe V, Barrio JR et al (2011) The merits of FDDNP-PET imaging in Alzheimer’s disease. J Alzheimers Dis 26:135–145
Sipos E, Kurunczi A, Kasza A et al (2007) Beta-amyloid pathology in the entorhinal cortex of rats induces memory deficits: implications for Alzheimer’s disease. Neuroscience 147:28–36
Sloan HL, Good M, Dunnett SB (2006) Double dissociation between hippocampal and prefrontal lesions on an operant delayed matching task and a water maze reference memory task. Behav Brain Res 171:116–126
St Clair D, Johnstone M (2018) Using mouse transgenic and human stem cell technologies to model genetic mutations associated with schizophrenia and autism. Philos Trans R Soc Lond B Biol Sci 373:pii:20170037
Steimer T (2011) Animal models of anxiety disorders in rats and mice: some conceptual issues. Dialogues Clin Neurosci 13:495–506
Stępnicki P, Kondej M, Kaczor AA (2018) Current concepts and treatments of Schizophrenia. Molecules 23. pii: E2087
Striedter GF (1998) Progress in the study of brain evolution: from speculative theories to testable hypotheses. Anat Rec 253:105–112
Sturchler-Pierrat C, Abramowski D, Duke M et al (1997) Two amyloid precursor protein transgenic mouse models with Alzheimer disease-like pathology. Proc Natl Acad Sci U S A 94:13287–13292
Sunderland T, Tariot PN, Weingartner H et al (1986) Pharmacologic modelling of Alzheimer’s disease. Prog Neuro-Psychopharmacol Biol Psychiatry 10:599–610
Swerdlow NR, Kuczenski R, Goins JC et al (2005) Neurochemical analysis of rat strain differences in the startle gating-disruptive effects of dopamine agonists. Pharmacol Biochem Behav 80:203–211
Swerdlow NR, Martinez ZA, Hanlon FM et al (2000) Toward understanding the biology of a complex phenotype: rat strain and substrain differences in the sensorimotor gating-disruptive effects of dopamine agonists. J Neurosci 20:4325–4336
Swerdlow NR, Shilling PD, Breier M et al (2012) Fronto-temporal-mesolimbic gene expression and heritable differences in amphetamine-disrupted sensorimotor gating in rats. Psychopharmacology 224:349–362
Sy M, Kitazawa M, LaFerla F (2011) The 3xTg-AD mouse model: reproducing and modulating plaque and tangle pathology. In: De Deyn PP, Van Dam D (eds) Animal models of dementia, 1st edn. Springer Science + Business Media, New York
Szabados T, Dul C, Majtényi K et al (2004) A chronic Alzheimer’s model evoked by mitochondrial poison sodium azide for pharmacological investigations. Behav Brain Res 154:31–40
Teipel SJ, Buchert R, Thome J et al (2011) Development of Alzheimer-disease neuroimaging-biomarkers using mouse models with amyloid-precursor protein-transgene expression. Prog Neurobiol 95:547–556
Tekirian TL, Cole GM, Russell MJ et al (1996) Carboxy terminal of beta-amyloid deposits in aged human, canine, and polar bear brains. Neurobiol Aging 17:249–257
Toledana A, Alvarez MI (2011) Lesion-induced vertebrate models of Alzheimer dementia. In: De Deyn PP, Van Dam D (eds) Animal models of dementia, 1st edn. Springer Science + Business Media, New York
Toyama H, Ye D, Ichise M et al (2005) PET imaging of brain with the beta-amyloid probe, [11C]6-OH-BTA-1, in a transgenic mouse model of Alzheimer’s disease. Eur J Nucl Med Mol Imaging 32:593–600
Trunnell ER (2019) Use of the forced swim test to assess “despair”. Brain Stimul pii: S1935-861X(19)30277-3
Tsai G, Coyle JT (2002) Glutamatergic mechanisms in schizophrenia. Annu Rev Pharmacol Toxicol 42:165–179
Tschaharganeh DF, Lowe SW, Garippa RJ, Livshits G (2016) Using CRISPR/Cas to study gene function and model disease in vivo. FEBS J 283:3194–3203
Tsukada H, Harada N, Nishiyama S et al (2000) Ketamine decreased striatal [(11)C]raclopride binding with no alterations in static dopamine concentrations in the striatal extracellular fluid in the monkey brain: multiparametric PET studies combined with microdialysis analysis. Synapse 37:95–103
Tulving E (1987) Multiple memory systems and consciousness. Hum Neurobiol 6:67–80
Uchida K, Yoshino T, Yamaguchi R et al (1995) Senile plaques and other senile changes in the brain of an American black bear. Vet Pathol 32:412–414
Urban N, Abi-Dargham A (2010) Neurochemical imaging in schizophrenia. Curr Top Behav Neurosci 4:215–242
Uylings HB, Groenewegen HJ, Kolb B (2003) Do rats have a prefrontal cortex? Behav Brain Res 146:3–17
Vale-Martínez A, Guillazo-Blanch G, Martí-Nicolovius M et al (2002) Electrolytic and ibotenic acid lesions of the nucleus basalis magnocellularis interrupt long-term retention, but not acquisition of two-way active avoidance, in rats. Exp Brain Res 142:52–66
Valzelli L (1973) The “isolation syndrome” in mice. Psychopharmacologia 31:305–320
van Berckel BN, Kegeles LS, Waterhouse R et al (2006) Modulation of amphetamine-induced dopamine release by group II metabotropic glutamate receptor agonist LY354740 in non-human primates studied with positron emission tomography. Neuropsychopharmacology 31:967–977
Van Dam D, De Deyn PP (2006) Drug discovery in dementia: the role of rodent models. Nat Rev Drug Discov 5:956–970
Van Dam D, De Deyn PP (2011a) The role of rodent models in the drug discovery pipeline for dementia. In: De Deyn PP, Van Dam D (eds) Animal models of dementia, 1st edn, Neuromethods series. Springer Science + Business Media, New York
Van Dam D, De Deyn PP (2011b) APP-based transgenic models: the APP23 model. In: De Deyn PP, Van Dam D (eds) Animal models of dementia, 1st edn. Springer Science + Business Media, New York
Van Dam D, De Deyn PP (2017) Non human primate models for Alzheimer's disease-related research and drug discovery. Expert Opin Drug Discov 12:187–200
Van Dam D, Van Dijck A, Janssen L et al (2013) Neuropeptides in Alzheimer’s disease: from pathophysiological mechanisms to therapeutic opportunities. Curr Alzheimer Res 10(5):449–468
Van Dam D, Vermeiren Y, Dekker AD et al (2016) Neuropsychiatric Disturbances in Alzheimer's Disease: What Have We Learned from Neuropathological Studies? Curr Alzheimer Res 13:1145–1164
van der Staay FJ (2006) Animal models of behavioral dysfunctions: basic concepts and classifications, and an evaluation strategy. Brain Res Rev 52:131–159
van der Staay FJ, Blokland A (1996) Behavioral differences between outbred Wistar, inbred Fischer 344, brown Norway, and hybrid Fischer 344 x brown Norway rats. Physiol Behav 60:97–109
van der Weyden L, Bradley A (2006) Mouse chromosome engineering for modeling human disease. Annu Rev Genomics Hum Genet 7:247–276
Van Dijck A, Vloeberghs E, Van Dam D et al (2008) Evaluation of the APP23-model for Alzheimer’s disease in the odour paired-associate test for hippocampus-dependent memory. Behav Brain Res 190:147–151
Varela MJ, Lage S, Caruncho HJ et al (2015) Reelin influences the expression and function of dopamine D2 and serotonin 5-HT2A receptors: a comparative study. Neuroscience 290:165–174
Varty GB, Walters N, Cohen-Williams M et al (2001) Comparison of apomorphine, amphetamine and dizocilpine disruptions of prepulse inhibition in inbred and outbred mice strains. Eur J Pharmacol 424:27–36
Vermeiren Y, Van Dam D, Aerts T et al (2014a) (2014) Brain region-specific monoaminergic correlates of neuropsychiatric symptoms in Alzheimer's disease. J Alzheimers Dis 41(3):819–833
Vermeiren Y, Van Dam D, Aerts T et al (2014b Dec) (2014) Monoaminergic neurotransmitter alterations in postmortem brain regions of depressed and aggressive patients with Alzheimer's disease. Neurobiol Aging 35(12):2691–2700
Vickers JC, Dickson TC, Adlard PA et al (2000) The cause of neuronal degeneration in Alzheimer’s disease. Prog Neurobiol 60:139–165
Vidal R, Ghetti B (2011) Characterization of amyloid deposits in neurodegenerative diseases. In: Manfredi G, Kawamata H (eds) Neurodegeneration: methods and protocols, 1st edn. Springer Science + Business Media, New York
Vloeberghs E, Van Dam D, Engelborghs S et al (2004) Altered circadian locomotor activity in APP23 mice: a model for BPSD disturbances. Eur J Neurosci 20:2757–2766
Vloeberghs E, Van Dam D, Franck F et al (2008) Altered ingestive behavior, weight changes, and intact olfactory sense in an APP overexpression model. Behav Neurosci 122:491–497
Vollenweider FX, Vontobel P, Oye I et al (2000) Effects of (S)-ketamine on striatal dopamine: a [11C]raclopride PET study of a model psychosis in humans. J Psychiatr Res 34:35–43
Voytko ML, Tinkler GP (2004) Cognitive function and its neural mechanisms in nonhuman primate models of aging, Alzheimer disease, and menopause. Front Biosci 9:1899–1914
Walsh DM, Selkoe DJ (2007) A beta oligomers—a decade of discovery. J Neurochem 101:1172–1184
Watanabe H (2017) [Development of SPECT Probes for In Vivo Imaging of β-Amyloid and Tau Aggregates in the Alzheimer's Disease Brain]. [Article in Japanese]. Yakugaku Zasshi 137:1361–1365
Weinstein JJ, Chohan MO, Slifstein M et al (2017) Pathway-Specific Dopamine Abnormalities in Schizophrenia. Biol Psychiatry 81:31–42
Weldon DT, Rogers SD, Ghilardi JR et al (1998) Fibrillar beta-amyloid induces microglial phagocytosis, expression of inducible nitric oxide synthase, and loss of a select population of neurons in the rat CNS in vivo. J Neurosci 18:2161–2173
Wenk GL, McGann K, Hauss-Wegrzyniak B et al (2003) The toxicity of tumor necrosis factor-alpha upon cholinergic neurons within the nucleus basalis and the role of norepinephrine in the regulation of inflammation: implications for Alzheimer’s disease. Neuroscience 121:719–729
Whitehouse PJ, Au KS (1986) Cholinergic receptors in aging and Alzheimer’s disease. Prog Neuro-Psychopharmacol Biol Psychiatry 10:665–676
Whitehouse PJ, Price DL, Struble RG et al (1982) Alzheimer’s disease and senile dementia: loss of neurons in the basal forebrain. Science 215:1237–1239
Williams NM, O’Donovan MC, Owen MJ (2005) Is the dysbindin gene (DTNBP1) a susceptibility gene for schizophrenia? Schizophr Bull 31:800–805
Willott JF, Carlson S, Chen H (1994) Prepulse inhibition of the startle response in mice: relationship to hearing loss and auditory system plasticity. Behav Neurosci 108:703–713
Willott JF, Tanner L, O’Steen J et al (2003) Acoustic startle and prepulse inhibition in 40 inbred strains of mice. Behav Neurosci 117:716–727
Wils H, Kleinberger G, Pereson S et al (2012) Cellular ageing, increased mortality and FTLD-TDP-associated neuropathology in progranulin knockout mice. J Pathol 228:67–76
Wimo A, Winblad B, Aguero-Torres H et al (2003) The magnitude of dementia occurrence in the world. Alzheimer Dis Assoc Disord 17:63–67
Winter C, Djodari-Irani A, Sohr R et al (2009) Prenatal immune activation leads to multiple changes in basal neurotransmitter levels in the adult brain: implications for brain disorders of neurodevelopmental origin such as schizophrenia. Int J Neuropsychopharmacol 12:513–524
Wirths O, Breyhan H, Cynis H et al (2009) Intraneuronal pyroglutamate-Abeta 3-42 triggers neurodegeneration and lethal neurological deficits in a transgenic mouse model. Acta Neuropathol 118(4):487–496
Wolf R, Dobrowolny H, Nullmeier S et al (2018) Effects of neonatal excitotoxic lesions in ventral thalamus on social interaction in the rat. Eur Arch Psychiatry Clin Neurosci 268:461–470
Wolf R, Matzke K, Paelchen K et al (2010) Reduction of Prepulse Inhibition (PPI) after neonatal excitotoxic lesion of the ventral thalamus in pubertal and adult rats. Pharmacopsychiatry 43:99–109
Wong DF, Kuwabara H1, Horti AG et al (2018) Brain PET Imaging of α7-nAChR with [18F]ASEM: Reproducibility, Occupancy, Receptor Density, and Changes in Schizophrenia. Int J Neuropsychopharmacol 21:656–667
Wong DF, Rosenberg PB, Zhou Y et al (2010) In vivo imaging of amyloid deposition in Alzheimer disease using the radioligand 18F-AV-45 (florbetapir [corrected] F 18). J Nucl Med 51:913–920
Woolley DG, Laeremans A, Gantois I et al (2013) Homologous involvement of striatum and prefrontal cortex in rodent and human water maze learning. Proc Natl Acad Sci U S A 110:3131–3136
Wu LS, Cheng WC, Hou SC et al (2010) TDP-43, a neuro-pathosignature factor, is essential for early mouse embryogenesis. Genesis 48:56–62
Yamada M, Chiba T, Sasabe J et al (2005) Implanted cannula-mediated repetitive administration of Abeta25-35 into the mouse cerebral ventricle effectively impairs spatial working memory. Behav Brain Res 164:139–146
Yang AC, Tsai SJ (2017) New targets for Schizophrenia treatment beyond the dopamine hypothesis. Int J Mol Sci 18. pii: E1689
Yochum CL, Bhattacharya P, Patti L et al (2010) Animal model of autism using GSTM1 knockout mice and early post-natal sodium valproate treatment. Behav Brain Res 210:202–210
Young JW, Minassian A, Paulus MP et al (2007) A reverse-translational approach to bipolar disorder: rodent and human studies in the Behavioral Pattern Monitor. Neurosci Biobehav Rev 31:882–896
Zhang S, Han D, Tan X et al (2012) Diagnostic accuracy of 18F-FDG and 11 C-PIB-PET for prediction of short-term conversion to Alzheimer’s disease in subjects with mild cognitive impairment. Int J Clin Pract 66:185–198
Download references
Acknowledgements
This work was supported by the Research Foundation Flanders (FWO), the Belgian Alzheimer Research Foundation—Stichting Alzheimer Onderzoek (SAO-FRA grants #2017/0025 and #2018/0027), the EU Joint Programme—Neurodegenerative Disease Research (JPND) project HEROES (ZonMW project 733051072), agreement between the Institute Born-Bunge and the University of Antwerp, the Medical Research Foundation Antwerp, the Thomas Riellaerts research fund and Neurosearch Antwerp.
Author information
Authors and affiliations.
Laboratory of Neurochemistry and Behaviour, Institute Born-Bunge, University of Antwerp, Wilrijk, Belgium
Debby Van Dam & Peter Paul De Deyn
Department of Neurology and Alzheimer Center Groningen, University of Groningen and University Medical Center Groningen (UMCG), Groningen, GZ, The Netherlands
Department of Neurology and Memory Clinic, Hospital Network Antwerp (ZNA), Middelheim and Hoge Beuken, Antwerp, Belgium
Peter Paul De Deyn
You can also search for this author in PubMed Google Scholar
Corresponding author
Correspondence to Debby Van Dam .
Editor information
Editors and affiliations.
Nuclear Medicine and Molecular Imaging, University Medical Center Groningen, Groningen, The Netherlands
Rudi A.J.O. Dierckx
Faculty of Electrical Engineering, Medical Engineering and Computer Science, Offenburg University, Offenburg, Germany
Andreas Otte
Erik F.J. de Vries
Nuclear Medicine and Molecular Imaging, University Medical Center, Groningen, The Netherlands
Aren van Waarde
Department of Radiology and Nuclear Medicine, Amsterdam University Medical Centers location VUmc, Amsterdam, The Netherlands
Adriaan A. Lammertsma
Rights and permissions
Reprints and permissions
Copyright information
© 2021 Springer Nature Switzerland AG
About this chapter
Van Dam, D., De Deyn, P.P. (2021). Animal Models for Brain Research. In: Dierckx, R.A., Otte, A., de Vries, E.F., van Waarde, A., Lammertsma, A.A. (eds) PET and SPECT of Neurobiological Systems. Springer, Cham. https://doi.org/10.1007/978-3-030-53176-8_1

Download citation
DOI : https://doi.org/10.1007/978-3-030-53176-8_1
Published : 30 September 2020
Publisher Name : Springer, Cham
Print ISBN : 978-3-030-53175-1
Online ISBN : 978-3-030-53176-8
eBook Packages : Medicine Medicine (R0)
Share this chapter
Anyone you share the following link with will be able to read this content:
Sorry, a shareable link is not currently available for this article.
Provided by the Springer Nature SharedIt content-sharing initiative
- Publish with us
Policies and ethics
- Find a journal
- Track your research
The browser you are using is no longer supported and for that reason you will not get the best experience when using our website.
You currently have JavaScript disabled in your web browser, please enable JavaScript to view our website as intended.
Groundbreaking research identifies what makes human brains – and humans – unique in the animal world
A neuroscientist at the University of Leicester has identified a fundamental difference between human and animal brains. This breakthrough, published today in the journal Cell , offers an explanation for what makes Homo sapiens so vastly different from even our nearest relatives. It could be the key to understanding human intelligence.
Professor Rodrigo Quian Quiroga, from our Department of Neuroscience, Psychology and Behaviour formerly discovered the so-called ‘ Jennifer Aniston neurons ’ or ‘concept cells’ – brain cells which fire repeatedly when the subject is shown different images of a particular person, or even that person’s name. The new article in Cell indicates that this type of neuron may be unique to humans. The research suggests that human memory differs from that of animals and hence is a potential root for high-level thoughts, based on concepts and abstractions, which are not found in even the most intelligent animals.
A great deal of neurological research has been done over the years on the brains of mice, rats and monkeys, which can be probed with electrodes to understand mechanisms of brain function. However, such research on humans is not allowed for obvious ethical reasons.
One of the very few situations in which an adult human has electrodes inserted into their brain is when they are being considered for surgery to treat epilepsy. This gives researchers such as Professor Quian Quiroga a unique opportunity to study the firing of individual human neurons in response to specific stimuli such as pictures and words.
Until now, it was believed that mammalian brains are all essentially similar across different species. Yet there must be some reason why humans evolved the way they did, differentiating themselves from other species. Other mammals can form memories, learn and think, but nowhere near the extent of humans. Even a young child rapidly outstrips other great apes in learning ability. Wherein lies the difference?
Professor Quian Quiroga proposes that ‘concept cells’ within the hippocampus, a part of the brain associated with memory, might be the answer. These cells have not been found in the hippocampus of monkeys or rats, suggesting that their presence can explain what makes humans different, leading to language, art, science and all the other manifestations of civilisation.
The key lies in the fact that a ‘concept cell’ fires to a specific subject, such as an actor or celebrity, irrespective of context. Whether the photograph of Jennifer Aniston shows her in character on a film set or walking the red carpet or relaxing at home, more or less the same neurons fire each time. They also fire when the subject sees or hears the actor’s name or makes an association, such as remembering seeing her in a particular place. Changing the clothes or context does not change the underlying ‘concept’ and so does not affect the neurons’ responses.
In contrast, studies in rats have shown that changes in their environment, such as a rearrangement of the arena where experiments take place, trigger a completely different set of neurons. The rat’s neurons do not respond to a slightly changed environment as the same place; changes in context change the neurons’ firing. Professor Quian Quiroga argues that concept cells in human brains, which store an abstract representation – the meaning of the stimulus, devoid of details and contextual information – might be key for making inferences, analogies and high-level associations that humans do naturally and effortlessly
This is the first time that a fundamental biological aspect has been identified which distinguishes human brains from those of other species.
Professor Quian Quiroga said: “The proposal that concept cells may be the key of human memory and our unique cognitive abilities is quite bold and at the same time fascinating. One just needs to realise that a chimpanzee’s brain is about a third of the size of a human brain to understand that there should be something radically different in the way the human brain functions. The idea that concept cells are key to human intelligence has wide reaching connotations to understand cognitive differences with other species and perhaps with artificial intelligence. Right or wrong, it will hopefully open new discussions about what makes us human that go beyond plain philosophy and are based on neuroscientific findings.”
Professor Ian Forsythe, Head of the Department of Neuroscience, Psychology and Behaviour at the University of Leicester, said: “Rodrigo Quian Quiroga’s research and ideas are challenging what we thought we knew about human cognition, and have far reaching consequences for understanding the brain and of what makes us human.”
- ‘Plugging in to Human Memory: Advantages, Challenges, and Insights from Human Single-Neuron Recordings’ doi: 10.1016/j.cell.2019.10.016
- Neuroscience
Related stories
£8.2m award from esprc to transform how technology critical materials are recycled and reused, university of leicester joins launch of amast network to battle amr in the agrifood system, over £600,000 for university of leicester to shrink ai algorithms for smarter spacecraft, national conference celebrates record attendance in leicester, space power: radioisotope thermoelectric generator aces vibration test, archaeology students from university of leicester unearth very big roman house in the country, space park leicester’s new satellite system monitoring real-time imagery of hurricane beryl.
- Brain Development
- Childhood & Adolescence
- Diet & Lifestyle
- Emotions, Stress & Anxiety
- Learning & Memory
- Thinking & Awareness
- Alzheimer's & Dementia
- Childhood Disorders
- Immune System Disorders
- Mental Health
- Neurodegenerative Disorders
- Infectious Disease
- Neurological Disorders A-Z
- Body Systems
- Cells & Circuits
- Genes & Molecules
- The Arts & the Brain
- Law, Economics & Ethics
- Neuroscience in the News
- Supporting Research
- Tech & the Brain
Animals in Research
- BRAIN Initiative
- Meet the Researcher
- Neuro-technologies
- Tools & Techniques
- Core Concepts
- For Educators
- Ask an Expert
- The Brain Facts Book

- BrainFacts/SfN
Basic neuroscience research in animal models is essential to understanding brain function and the thousands of brain diseases and disorders that affect both humans and animals. Treatments for stroke, depression, and drug addiction are just a few examples of developments made by this research. Learn more .
An interactive brain map that you can rotate in a three-dimensional space.
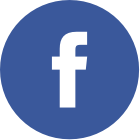
SUPPORTING PARTNERS
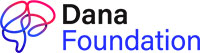
- Privacy Policy
- Accessibility Policy
- Terms and Conditions
- Manage Cookies
Some pages on this website provide links that require Adobe Reader to view.
Thank you for visiting nature.com. You are using a browser version with limited support for CSS. To obtain the best experience, we recommend you use a more up to date browser (or turn off compatibility mode in Internet Explorer). In the meantime, to ensure continued support, we are displaying the site without styles and JavaScript.
- View all journals
- Explore content
- About the journal
- Publish with us
- Sign up for alerts
- NEWS FEATURE
- 03 August 2022
Hybrid brains: the ethics of transplanting human neurons into animals
- Kendall Powell 0
Kendall Powell is a science journalist in Boulder, Colorado.
You can also search for this author in PubMed Google Scholar
Human neurons made from stem cells (green) two weeks after being transplanted into a mouse brain. Credit: Pierre Vanderhaeghen
In a darkened room in a laboratory in London, a group of students and researchers watch a clump of human brain cells settle into their new home: a living mouse brain. On a computer monitor next to a microscope, the human cells light up in flashes of simultaneous activity. Over time, the cells sprout new connections a few centimetres long, and form networks with each other. It’s captivating viewing for his students, says Vincenzo De Paola, who runs the lab at Imperial College London. “It’s all they want to do. I can’t tear them away,” he says.
Access options
Access Nature and 54 other Nature Portfolio journals
Get Nature+, our best-value online-access subscription
24,99 € / 30 days
cancel any time
Subscribe to this journal
Receive 51 print issues and online access
185,98 € per year
only 3,65 € per issue
Rent or buy this article
Prices vary by article type
Prices may be subject to local taxes which are calculated during checkout
Nature 608 , 22-25 (2022)
doi: https://doi.org/10.1038/d41586-022-02073-4
Mosier, D. E., Gulizia, R. J., Baird, S. M. & Wilson, D. B. Nature 335 , 256–259 (1988).
Article PubMed Google Scholar
Espuny-Camacho, I. et al. Neuron 77 , 440–456 (2013).
Crane, A. T. et al. Stem Cell Rep. 15 , 804–810 (2020).
Article Google Scholar
Linaro, D. et al. Neuron 104 , 972–986 (2019).
Espuny-Camacho, I. et al. Neuron 93 , 1066–1081 (2017).
Real, R. et al. Science 362 , eaau1810 (2018).
Lancaster, M. et al. Nature 501 , 373–379 (2013).
Mansour, A. A. et al. Nature Biotechnol. 36 , 432–441 (2018).
Tan, T. et al. Cell 184 , 2020–2032 (2021).
Yamaguchi, T. et al. Nature 542 , 191–196 (2017).
Chang, A. N. et al. Nature 563 , 126–130 (2018).
Download references
Reprints and permissions
Related Articles
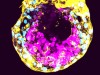
- Developmental biology
- Neuroscience
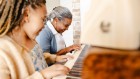
Memory for music doesn’t diminish with age
News 24 JUL 24
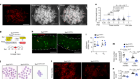
Clonal inactivation of TERT impairs stem cell competition
Article 17 JUL 24
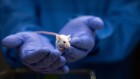
Mice live longer when inflammation-boosting protein is blocked
News 17 JUL 24
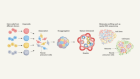
Chimeric brain organoids capture human genetic diversity
News & Views 26 JUN 24
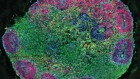
These 3D model brains with cells from several people are first of their kind
News 26 JUN 24
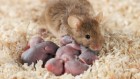
These brain cells help days-old mice to bond with mum
News 25 JUL 24
Neural circuit basis of placebo pain relief
Article 24 JUL 24
Stadtman Investigator Search 2024-2025
Stadtman Investigator Search 2024-2025 Deadline: September 30, 2024 The National Institutes of Health, the U.S. government’s premier biomedical and...
Bethesda, Maryland
National Institute of Health- Office of Intramural Research
Research Manager, Open Access
The Research Manager is a great opportunity for someone with experience of policy and open access to join Springer Nature.
London, Berlin or New York (Hybrid Working)
Springer Nature Ltd
Postdoctoral Research Scientist
Computational Postdoctoral Research Scientist in Neurodegenerative Disease
New York City, New York (US)
Columbia University Department of Pathology
Associate or Senior Editor (Chemical Engineering), Nature Sustainability
Associate or Senior Editor (Chemical engineering), Nature Sustainability Locations: New York, Philadelphia, Berlin, or Heidelberg Closing date: Aug...
Postdoctoral Research Scientist - Mouse Model
The Stavros Niarchos Foundation Center for Precision Psychiatry & Mental Health is seeking a Postdoctoral Research Scientist for the Mouse Model.
Columbia University Irving Medical Center / Vagelos College of Physicians and Surgeons
Sign up for the Nature Briefing newsletter — what matters in science, free to your inbox daily.
Quick links
- Explore articles by subject
- Guide to authors
- Editorial policies
- How to Contact Us
- Library & Collections
- Business School
- Things To Do
/prod01/prodbucket01/media/durham-university/research-/73895.webp)
Bigger animals don’t always have the biggest brains relative to body size – new research
- Thought Leadership
- Anthropology
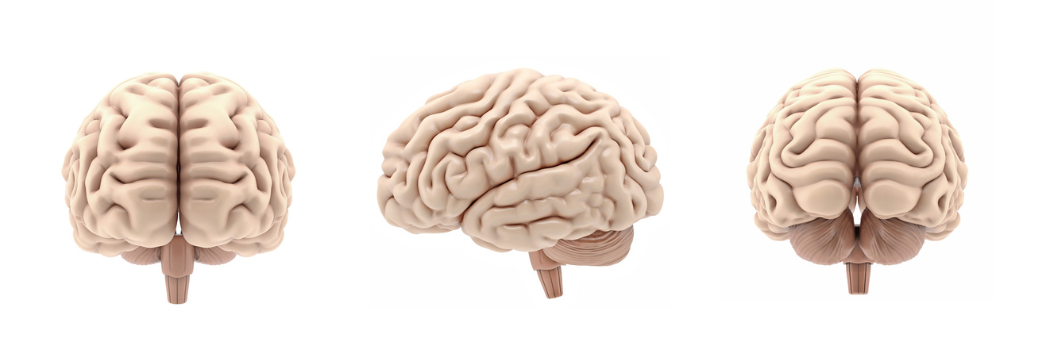
Scientists have long believed that big animals will tend to have big brains, but a new study involving Professor Robert Barton, from our Department of Anthropology, has found that may not be the case.
Scientists have long believed that, generally speaking, the bigger an animal is, the bigger its brain. But our recent study challenges the nature of that linear view and reveals new insights about how brain size and body size have evolved together.
There is astounding diversity in the shape, size and internal structure of the brain among different animals. While the size of the brain usually correlates with cognitive abilities , its variation is strongly linked to the size of the animal itself.
As an animal gets larger, its brain size increases as well, though not in direct proportion – it is not a one-to-one relationship. This phenomenon is called allometric scaling .
Body size in mammals ranges from tiny bumblebee bats weighing in at less than two grams right up to the largest animal that has ever lived, the several-tonne blue whale . So we need to understand how body size and brain size have evolved together to say anything meaningful about how variation in brain size developed.
This is what our recent study tried to do. We applied powerful computational analysis to a dataset spanning over 1,500 species of mammal and their brain sizes. We asked how has the size of brains evolved in relation to the size of the animal?
Our results revealed a surprisingly simple pattern of evolutionary change between brain and body size. We found that the relationship between brain and body size is not linear, as previously assumed , but curved. It plateaus once body size reaches a certain threshold.
Previously, researchers had scratched their heads over the great variability in the brain and body size relationship. For example, the relationship appeared much steeper in some groups of animals than others (such as elephants and their cousins in purple compared with primates in pink).
The relationship between brain and body size is less strong among species of the same family (such as the ape family Hominidae, or the dog and wolf family Canidae) than it is among species of the same order (for example primates or carnivores).
We took another look at the data to investigate why brain size diminishes in some big animals. In our paper, we demonstrated – to our surprise – that this constraint is not explained by the high energy cost of maintaining a large brain. ( About 20% of all energetic expenditure in humans is dedicated to the brain.) Nor is it linked to neuron density, which may allow enhanced processing power without the need for a bigger brain.
So, the processes behind this aspect of our findings remain a mystery – for now.
However, our study allowed us to which identify mammal species are outliers to the brain-body size relationship. To do this, we used an analysis method that identified species and lineages in which there have been intensely rapid changes in brain size.
Among these rapidly evolving lineages is our own species, Homo sapiens . We found that our large brains developed at a rate of evolution more than 20 times faster than the average rate of all other mammal species, resulting in the massive brains that characterise humanity today.
The fact that humans are outliers to a mammal-wide pattern fits with our ability to use tools, build houses, make music and live in complex societies. But humans are not the only species to stray from the curve.
We found many species throughout the mammalian tree of life which have rapidly increased their brain size (relative to their body size), including several species noted for their keen intelligence such as rats, bears, dolphins and elephants. But also others like wallabies, marmots and quolls.
Conversely, we observed a massive acceleration in rate towards smaller brain sizes when bats first evolved. But brain size has evolved minimally and slowly over the following 50 million years or so of their evolution. This could indicate some sort of constraint acting on brain size in bats, perhaps imposed by flight.
We found rapid bursts of change in the relative size of an animal’s brain most frequently in carnivores, rodents and primates. In these three groups, these rapid changes almost always result in proportionally larger brains.
This principle, known as the Marsh-Lartet rule, would suggest that relative brain size tends to increase over time in mammal evolution. But our models suggest that this is not a universal phenomenon observed across all species.
The three mammal groups where the trend does apply have profoundly diverse behaviour and ecology even among close relatives (for example pandas and grizzly bears, or porcupines and guinea pigs). They include some of the most social animals such as humans, mole-rats and meerkats.
Primate brain size has always been growing. The earliest primates had brains about 0.5% of their body size . Eventually, increasing brain size eventually culminated in our own large brains (about 2% of our body size).
Our data shows that sometimes it really is a simple explanation that can explain the complexities observed in nature. However, natural selection is inherently complex. Like any rule, the curved relationship between brain and body size has been repeatedly broken by animals who have evolved ahead of the curve.
Find out more
This article is republished from The Conversation under a Creative Commons license. Read the original article .
Read more about Professor Robert Barton .
- Find out more about this research on the Durham University webpages .
Our Department of Anthropology is ranked 28th in the QS World University Rankings by Subject 2024.
Visit our Anthropology webpages for more information on our undergraduate and postgraduate programmes.
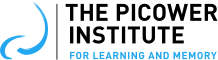
HHMI honor will advance Flavell’s studies of how internal brain states arise and affect behavior
In animals from worms to humans, feelings and drives such as hunger or sleepiness influence behavior. With flexible, long-term support as a newly named Howard Hughes Medical Institute Investigator, Steve Flavell will advance his lab’s studies of exactly how that happens.
The things we do for love. The mistakes we make when we’re tired or rushed. The angry words we wish we could take back. The times we jump for joy.
The episodes when our internal emotions and motivations affect our behavior provide some of our strongest and most personal experiences with our selves. But as intensely felt as these times and underlying states can be, they have been difficult for neuroscientists to define and explain. Related disorders such as depression can therefore be harder to treat. As a newly named HHMI Investigator , Associate Professor Steve Flavell directs a research program in a much simpler organism, the roundworm C. elegans , that he hopes will prove foundational to building an understanding of how internal states arise and influence behavior in nervous systems in general.
“I think that it should be possible to define the basis of internal states in C. elegan s in concrete terms ,” Flavell said. “If we can build a thread of understanding from the molecular architecture of neuromodulatory systems, to changes in brain-wide activity, to state-dependent changes in behavior, then I think we’ll be in a much better place as a field to think about the basis of brain states in more complex animals.”
The long-term impact would not only be a better understanding of the link between brain activity and behavior, but also improved interventions for psychiatric disorders. Many psychiatric medicines target neuromodulatory brain chemicals, such as serotonin, that lie at the heart of the dynamics Flavell is investigating.
Indeed, these goals have guided Flavell’s lab in The Picower Institute for Learning and Memory and the Department of Brain and Cognitive Sciences at MIT ever since he established it in 2016, but now with the HHMI’s long-term, flexible support he can address the full set of scientific challenges necessary to answer these questions. HHMI’s “people, not projects” approach in supporting the investigators it selects provides generous, renewable funding that provides the flexibility to let investigators go where ever their scientific questions take them.
“This allows us to think about these scientific problems in a manner that embraces their full complexity,” said Flavell, who is one of four MIT faculty members and 26 scientists around the country to be named HHMI Investigators this year.
A multidisciplinary research program
C. elegans is an animal that is simple enough to be studied from the molecular to whole-brain scale, but deceptively complex nonetheless. Though the entire connectome, or wiring diagram, among its 302 neurons has been fully mapped and its genes are readily manipulable in lab experiments, the worm nevertheless displays a remarkable array of flexible behaviors that often employ the same molecular machinery found in humans, including neuromodulatory chemicals such as serotonin and dopamine. In all, the worm has more than100 different neuromodulators that might enable its internal states to change how it behaves.
In recent years, Flavell’s lab has published studies showing how specific neural circuits, employing neuromodulators, integrate inputs such as sensory information and internal states to produce flexible behaviors. In two papers last year, his lab and collaborators blended techniques ranging from genetic and molecular manipulations, to imaging of behavior and brain-wide neural activity, to computational modeling to produce major brain-wide insights. In one study, the team built a model that can predict how a majority of the worm’s neurons encode and represent its behaviors, and found that about 30 percent of the worm’s neurons remain flexible to account for different behavioral situations. In another paper the scientists thoroughly mapped the worm’s six distinct serotonin receptors (humans have 14) across each neuron in its brain, and showed how serotonin release acts on each of them to affect feeding and locomotion.
Framework for the future
One of the main new thrusts of the lab’s work, Flavell said, will be to build on those achievements by constructing a new generation of computational models that predict how every neuron responds to a wider range of internal states in the worm.
Another new area of work will delve deeper into how neuromodulators affect the animal’s internal states, ranging from studies of how these chemicals are released and diffuse to reach their targets and studies of how they act in combination, Flavell said.
A third major initiative in the lab will tackle the question of how specific features of the connectome – aspects of nervous system wiring – control internal states.
A recognition of the whole lab
The HHMI award’s support doesn’t just open up these new opportunities for Flavell, but for his whole lab. As a team of postdocs, graduate students, technicians and other trainees, Flavell said, his lab will now have greater flexibility to consider the biggest questions and implications of their results and to take whatever approaches are needed to investigate them.
In that spirit, as Flavell reflected on being named an HHMI Investigator, which is one of the most prestigious honors in life sciences research, he said he thought of the many contributions former and current members of his lab have made over the years.
“Many of my scientific heroes are HHMI Investigators,” Flavell said. “The idea of being included in that group is humbling. But my main reaction is that I just feel extraordinarily proud of the current and former members of my lab. This is really their achievement.”
Related Articles
Livestreaming the brain.
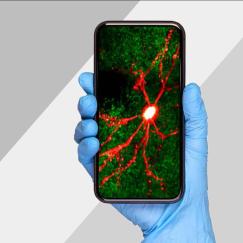
Study shows how a single neuron’s parallel outputs can coordinate many aspects of behavior
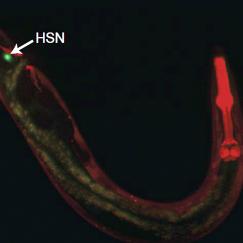
Cracking the code that relates brain and behavior in a simple animal
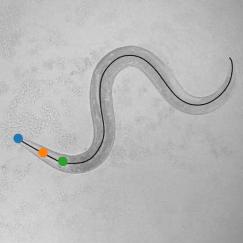
Petite & Profound
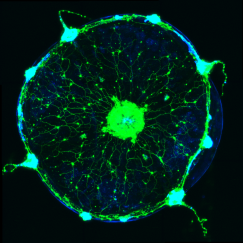
Suggestions or feedback?
MIT News | Massachusetts Institute of Technology
- Machine learning
- Sustainability
- Black holes
- Classes and programs
Departments
- Aeronautics and Astronautics
- Brain and Cognitive Sciences
- Architecture
- Political Science
- Mechanical Engineering
Centers, Labs, & Programs
- Abdul Latif Jameel Poverty Action Lab (J-PAL)
- Picower Institute for Learning and Memory
- Lincoln Laboratory
- School of Architecture + Planning
- School of Engineering
- School of Humanities, Arts, and Social Sciences
- Sloan School of Management
- School of Science
- MIT Schwarzman College of Computing
Study reveals how an anesthesia drug induces unconsciousness
Press contact :, media download.
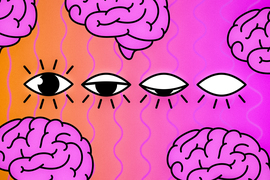
*Terms of Use:
Images for download on the MIT News office website are made available to non-commercial entities, press and the general public under a Creative Commons Attribution Non-Commercial No Derivatives license . You may not alter the images provided, other than to crop them to size. A credit line must be used when reproducing images; if one is not provided below, credit the images to "MIT."
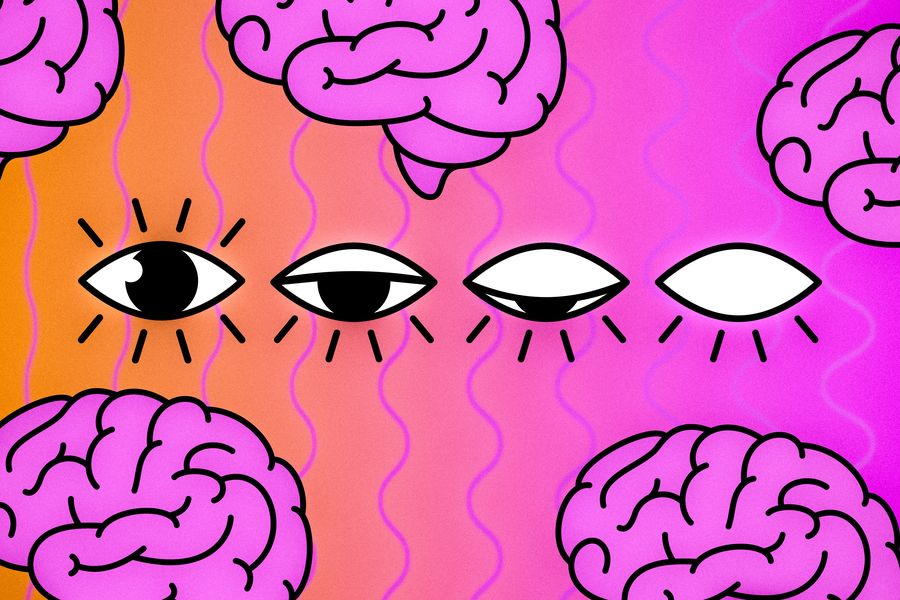
Previous image Next image
There are many drugs that anesthesiologists can use to induce unconsciousness in patients. Exactly how these drugs cause the brain to lose consciousness has been a longstanding question, but MIT neuroscientists have now answered that question for one commonly used anesthesia drug.
Using a novel technique for analyzing neuron activity, the researchers discovered that the drug propofol induces unconsciousness by disrupting the brain’s normal balance between stability and excitability. The drug causes brain activity to become increasingly unstable, until the brain loses consciousness.
“The brain has to operate on this knife’s edge between excitability and chaos. It’s got to be excitable enough for its neurons to influence one another, but if it gets too excitable, it spins off into chaos. Propofol seems to disrupt the mechanisms that keep the brain in that narrow operating range,” says Earl K. Miller, the Picower Professor of Neuroscience and a member of MIT’s Picower Institute for Learning and Memory.
The new findings, reported today in Neuron , could help researchers develop better tools for monitoring patients as they undergo general anesthesia.
Miller and Ila Fiete, a professor of brain and cognitive sciences, the director of the K. Lisa Yang Integrative Computational Neuroscience Center (ICoN), and a member of MIT’s McGovern Institute for Brain Research, are the senior authors of the new study. MIT graduate student Adam Eisen and MIT postdoc Leo Kozachkov are the lead authors of the paper.
Losing consciousness
Propofol is a drug that binds to GABA receptors in the brain, inhibiting neurons that have those receptors. Other anesthesia drugs act on different types of receptors, and the mechanism for how all of these drugs produce unconsciousness is not fully understood.
Miller, Fiete, and their students hypothesized that propofol, and possibly other anesthesia drugs, interfere with a brain state known as “dynamic stability.” In this state, neurons have enough excitability to respond to new input, but the brain is able to quickly regain control and prevent them from becoming overly excited.
Previous studies of how anesthesia drugs affect this balance have found conflicting results: Some suggested that during anesthesia, the brain shifts toward becoming too stable and unresponsive, which leads to loss of consciousness. Others found that the brain becomes too excitable, leading to a chaotic state that results in unconsciousness.
Part of the reason for these conflicting results is that it has been difficult to accurately measure dynamic stability in the brain. Measuring dynamic stability as consciousness is lost would help researchers determine if unconsciousness results from too much stability or too little stability.
In this study, the researchers analyzed electrical recordings made in the brains of animals that received propofol over an hour-long period, during which they gradually lost consciousness. The recordings were made in four areas of the brain that are involved in vision, sound processing, spatial awareness, and executive function.
These recordings covered only a tiny fraction of the brain’s overall activity, so to overcome that, the researchers used a technique called delay embedding. This technique allows researchers to characterize dynamical systems from limited measurements by augmenting each measurement with measurements that were recorded previously.
Using this method, the researchers were able to quantify how the brain responds to sensory inputs, such as sounds, or to spontaneous perturbations of neural activity.
In the normal, awake state, neural activity spikes after any input, then returns to its baseline activity level. However, once propofol dosing began, the brain started taking longer to return to its baseline after these inputs, remaining in an overly excited state. This effect became more and more pronounced until the animals lost consciousness.
This suggests that propofol’s inhibition of neuron activity leads to escalating instability, which causes the brain to lose consciousness, the researchers say.
Better anesthesia control
To see if they could replicate this effect in a computational model, the researchers created a simple neural network. When they increased the inhibition of certain nodes in the network, as propofol does in the brain, network activity became destabilized, similar to the unstable activity the researchers saw in the brains of animals that received propofol.
“We looked at a simple circuit model of interconnected neurons, and when we turned up inhibition in that, we saw a destabilization. So, one of the things we’re suggesting is that an increase in inhibition can generate instability, and that is subsequently tied to loss of consciousness,” Eisen says.
As Fiete explains, “This paradoxical effect, in which boosting inhibition destabilizes the network rather than silencing or stabilizing it, occurs because of disinhibition. When propofol boosts the inhibitory drive, this drive inhibits other inhibitory neurons, and the result is an overall increase in brain activity.”
The researchers suspect that other anesthetic drugs, which act on different types of neurons and receptors, may converge on the same effect through different mechanisms — a possibility that they are now exploring.
If this turns out to be true, it could be helpful to the researchers’ ongoing efforts to develop ways to more precisely control the level of anesthesia that a patient is experiencing. These systems, which Miller is working on with Emery Brown, the Edward Hood Taplin Professor of Medical Engineering at MIT, work by measuring the brain’s dynamics and then adjusting drug dosages accordingly, in real-time.
“If you find common mechanisms at work across different anesthetics, you can make them all safer by tweaking a few knobs, instead of having to develop safety protocols for all the different anesthetics one at a time,” Miller says. “You don’t want a different system for every anesthetic they’re going to use in the operating room. You want one that’ll do it all.”
The researchers also plan to apply their technique for measuring dynamic stability to other brain states, including neuropsychiatric disorders.
“This method is pretty powerful, and I think it’s going to be very exciting to apply it to different brain states, different types of anesthetics, and also other neuropsychiatric conditions like depression and schizophrenia,” Fiete says.
The research was funded by the Office of Naval Research, the National Institute of Mental Health, the National Institute of Neurological Disorders and Stroke, the National Science Foundation Directorate for Computer and Information Science and Engineering, the Simons Center for the Social Brain, the Simons Collaboration on the Global Brain, the JPB Foundation, the McGovern Institute, and the Picower Institute.
Share this news article on:
Press mentions.
MIT scientists have discovered how propofol, a commonly used anesthetic, induces unconsciousness, reports Adam Kovac for Gizmodo . “The new research indicates that [propofol] works by interfering with a brain’s ‘dynamic stability’ – a state where neurons can respond to input, but the brain is able to keep them from getting too excited,” explains Kovac.
IFL Science
MIT researchers have discovered how propofol, a commonly used anesthetic, works on the brain, reports Francesca Benson for IFL Science . The research studied “the differences between an awake brain and one under anesthesia by looking at the stability of the brain’s activity,” writes Bensen.
Previous item Next item
Related Links
- Earl Miller
- Department of Brain and Cognitive Sciences
- Picower Institute
- McGovern Institute
Related Topics
- Brain and cognitive sciences
- National Institutes of Health (NIH)
- National Science Foundation (NSF)
Related Articles
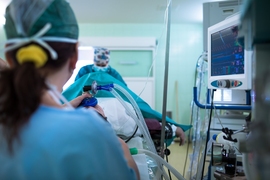
Anesthesia technology precisely controls unconsciousness in animal tests
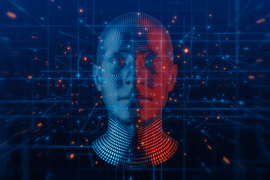
The brain may learn about the world the same way some computational models do
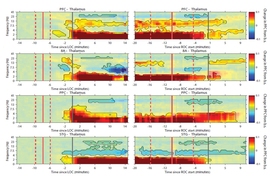
Anesthesia doesn't simply turn off the brain — it changes its rhythms
More mit news.

Edgerton Center hosts workshop for deaf high school students in STEM
Read full story →

MIT Global SCALE Network expands by adding center at Loughborough University
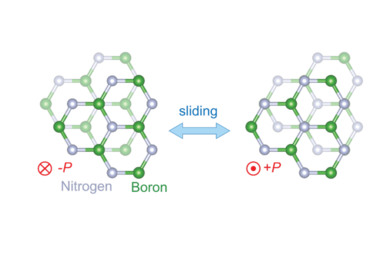
New transistor’s superlative properties could have broad electronics applications
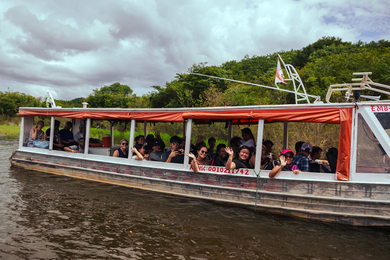
When learning at MIT means studying thousands of miles away
Flying high to enable sustainable delivery, remote care
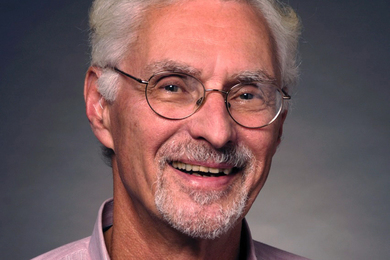
Professor Emeritus Ralph Gakenheimer, mobility planner and champion of international development, dies at 89
- More news on MIT News homepage →
Massachusetts Institute of Technology 77 Massachusetts Avenue, Cambridge, MA, USA
- Map (opens in new window)
- Events (opens in new window)
- People (opens in new window)
- Careers (opens in new window)
- Accessibility
- Social Media Hub
- MIT on Facebook
- MIT on YouTube
- MIT on Instagram
- Jul 25 2024
Sanders-Brown research shows clear connection between socialization, enrichment and brain health

Researchers from the University of Kentucky’s Sanders-Brown Center on Aging and the University of California Irvine are some of the first to show socialization and enrichment are good for aging brains.
The collaborative study was recently published in the Journal of Neuroscience . Imaging from the work was so compelling it was chosen as the cover art for the issue.
The project began in 2019 with a $1.2 million grant from the National Institutes of Health’s National Institute on Aging . Researchers’ goal was to determine whether cognitive loss and other signs of neural dysfunction could be slowed down in aging dogs using drugs that reduce neuroinflammation and synaptic communication deficits.
“This study has great parallels to human health and disease,” said Chris Norris, Ph.D., one of the principal investigators of the study. He is a professor of pharmacology and nutritional sciences in the UK College of Medicine and Sanders-Brown faculty. “Staying socially connected, exercising and keeping your brain engaged with stimulating activities may provide substantial protection against the detrimental effects of aging and age-related diseases like Alzheimer’s.”
Synaptic communication deficits refer to problems in the way nerve cells (neurons) in the brain communicate with each other. Neurons send messages to one another through connections called synapses. If these connections aren’t working properly, it can lead to difficulties in how the brain processes information. This can affect various brain functions, such as learning, memory and overall cognitive abilities. These deficits are often linked to neurological conditions like autism, schizophrenia and Alzheimer’s disease.
“Dogs were chosen because they live in similar environments to humans, have similar metabolism and can perform complex learning tasks," said Norris. "Also importantly, beginning in middle age, some beagles develop Alzheimer’s-like amyloid plaques in the brain connected with cognitive loss — similar to humans.”
For the study, researchers worked with healthy middle-aged dogs that received daily oral doses of either a placebo or experimental drugs over three years. Nine years of age in a dog is comparable to 60 years in a human.
During this time, the dogs engaged in daily socialization with human caregivers and other dogs in a highly enriched environment that included toys, physical exercise and exploration.
The dogs were also routinely tested on several learning and memory tasks. They were assessed at the beginning of the study and then after each year of treatment researchers looked at the dogs’ brains using magnetic resonance imaging (MRI), similar to what is used in humans to look at changes in brain size.
“Typically, what we see in both dogs and humans is that brains begin to shrink with old age, and especially with Alzheimer’s pathology. What we found really surprised us,” said Norris.
Contrary to what the researchers predicted would happen, the drug treatments had little effect on brain size during the study. But the surprise finding was that one brain region — the hippocampus — increased in size over the three-year period for nearly all dogs.
“This was very unusual. The hippocampus is critical to learning and memory and is one of the first brain regions to show structural and functional changes, including atrophy or shrinking, during Alzheimer’s disease,” said Norris. “The best explanation for why the hippocampus grew in our dogs was because of the high levels of behavioral enrichment and socialization they were exposed to.”
Though the research team was a little disappointed that drug treatments failed to affect age-related brain size measures, they were nonetheless excited about the data and believe the implications will be highly valuable to both the research community and the general public.
“Many of us are dog owners and we love our dogs!" said Norris. "Just like humans, dogs can develop cognitive deficits when they get older. They may pace around a lot, become confused, seem to forget where they are and some may show ‘personality’ changes. Our study shows that playing with your dog, providing regular exercise and social interactions with other dogs can help improve brain health, which could improve quality of life in your dog’s later years.”
The work is among the first studies to show beneficial effects of socialization and behavioral enrichment on the brain size in a mammal model with many similarities to humans.
Socialization, activity engagement and enriched environments are areas that researchers at Sanders-Brown often encourage when they hold workshops with the community and in conducting clinical trials with humans.
“Keeping your mind and body actively engaged in various activities, including socialization, exercise and cognitively stimulating tasks, can help keep the brain sharp as we age,” said Elizabeth Rhodus, Ph.D., an assistant professor in the College of Medicine and faculty at Sanders-Brown. “Whether it is a monthly dinner with friends, a new card club or volunteering for change, engagement and socialization are key ingredients to a long and healthy lifestyle.”
Rhodus is currently leading a clinical trial with individuals with moderate to severe cognitive loss and their caregivers which specifically addresses socialization, activity engagement and environmental enrichment. A recent participant caregiver acknowledged the importance of this type of research as she reflected on the notable changes she and her mother experienced.
“I’ve gotten far more out of it than I even dreamed I would,” she shared. “I feel like things have really improved the quality of our life and the quality of my life, and it’s been very good. [My mother] even sang… I have heard her sing, but it has been decades. She was singing to herself… when the song got done and she was done singing, she just laughed and laughed like she just had the greatest time. It’s kind of fun.”
Norris and the rest of the collaborative teams believe this research will have a direct impact on patient care even if it’s not in the form of a potential drug therapy. Socialization and behavioral enrichment are something all of us can do as we get older. It’s a highly practical, relatively inexpensive and non-invasive way to ward off the effects of aging and Alzheimer’s.
“It is something that’s been talked about before, but now we have clear proof of it. Bottom line is socialization, behavioral enrichment and environmental enrichment are good for your brain,” said Norris.
Research reported in this publication was supported by the National Institute on Aging of the National Institutes of Health under Award Number R01AG056998. The content is solely the responsibility of the authors and does not necessarily represent the official views of the National Institutes of Health.
Words: Hillary Smith (Public Relations & Strategic Communication) Cover image: Jessica Noche
- Neuroscience
- Alzheimer's Disease
You might also like...

More from this series Research Priorities - Neuroscience


An official website of the United States government
Here's how you know
The .gov means it’s official. Federal government websites often end in .gov or .mil. Before sharing sensitive information, make sure you’re on a federal government site.
The site is secure. The https:// ensures that you are connecting to the official website and that any information you provide is encrypted and transmitted securely.
NIH BRAIN Initiative at 10: Q&A with the NIA director July 24, 2024

Originally posted by the NIH BRAIN Blog in recognition of the 10-year anniversary of The BRAIN Initiative .
How has NIA participated in the NIH BRAIN Initiative?
NIA is the primary U.S. federal agency that supports and conducts research on brain aging and Alzheimer’s disease and related dementias. We are proud to be a part of this BRAIN Initiative that has achieved remarkable success over the past 10 years. In fiscal year 2024, NIA has contributed nearly $10 million to fund combined research grants and contracts to BRAIN Initiative efforts. Alongside many of our NIH colleagues, several NIA staff and I are eager to help shape an innovative road map for future collaborative BRAIN Initiative research, funding opportunities, and scientific workshops — in particular, those that expand understanding of the roles of aging and dementia on the brain.
What major BRAIN-funded scientific advancements or conversations has NIA been part of?
Mapping the brain’s cellular and molecular pathways can help provide a framework for better understanding normal brain function as well as how brain diseases like dementia develop. Today, through BRAIN Initiative investments, the BRAIN Initiative Cell Census Network and the BRAIN Initiative Cell Atlas Network are giving us high-resolution atlases. These powerful collections of precise maps can define and characterize the diversity of brain cell types and guide a framework to study brain function and disorders in animals and humans. One tremendous example is the Alzheimer’s Disease Multimodal Atlas Projects (AD-MAP) and the Seattle Alzheimer’s Disease Brain Cell Atlas (SEA-AD) . These collaborations with BRAIN are identifying brain cell types important in Alzheimer’s. What we — and the entire scientific community — learn from these powerful resources will deepen our understanding of the molecular and cellular pathways involved in Alzheimer’s as it progresses from early-stage to severe disease.
Beyond identifying brain cells and pathways involved in dementia, it is critical to be able to target brain cells to test potential therapies. To help more precisely monitor and modify brain cells for research purposes, NIA is building on the Armamentarium for Precision Brain Cell Access and other new Tools and Technologies for Brain Cells and Circuits emerging from BRAIN. These efforts will provide the next generation of targeted treatments that go beyond managing symptoms as is now mainly the case.
How do NIA’s research priorities align with the NIH BRAIN Initiative?
The BRAIN Initiative is a natural partner for NIA. For example, BRAIN aligns with our strategic research goal to improve understanding of the aging brain and support evidence-based strategies to maintain and enhance cognitive, emotional, sensory, and motor function. The BRAIN Initiative is accelerating the development of innovative neurotechnologies to probe brain function spatially and over time. This new and dynamic approach is a game-changer, enabling analysis of the entire brain, including the contribution of individual cells and neural circuits. This knowledge will help us understand both normal cognitive aging as well as the roots of neurodegenerative conditions including but not limited to Alzheimer’s and related dementias.
How has the BRAIN Initiative advanced or shaped our mission?
NIA is proud to be part of the BRAIN Initiative, and resources such as AD-MAP and SEA-AD are designed to help shape a future of innovation that will benefit older adults across our nation as well as globally. In addition to the critical tools we are developing, our partnership with BRAIN also aligns with NIA’s focus on building a strong, diverse scientific workforce in neuroscience and aging. For example, NIA participates in the BRAIN Initiative Advanced Career Transition Award to Promote Diversity (K99/R00) . Through this funding opportunity, NIA enables postdoctoral scientists whose research falls within the priority areas of BRAIN to transition into their first faculty position and launch independent careers. The program is designed to be more flexible than traditional NIH K99/R00 awards, an important characteristic for aging research, which can take many years to complete.
We look forward to future decades of continued progress and partnership with the BRAIN Initiative as it continues to deliver on its promise to revolutionize neuroscience research and bring cures for brain disorders.
Sign up for e-alerts about Inside NIA: A Blog for Researchers
Add new comment.
A red asterisk ( * ) indicates a required field.
- Allowed HTML tags: <p> <br>
- No HTML tags allowed.
- Lines and paragraphs break automatically.
- Web page addresses and email addresses turn into links automatically.
nia.nih.gov
An official website of the National Institutes of Health

IMAGES
VIDEO
COMMENTS
"Previous comparative studies established that the human brain is built like other mammalian brains, so we were surprised to find strong evidence that human neurons are special," says former MIT graduate student Lou Beaulieu-Laroche. Beaulieu-Laroche is the lead author of the study, which appears today in Nature. A building plan
The brain of any animal is constantly bombarded with information about the creature's environment from sensory organs such as the eyes, ears, nose or skin. All of this information is initially ...
Why Animals Are Vital to Brain Research. The brain is incredibly complex, and animal models offer a way to study all of its intricacies. The brains of ferrets (above) are currently being used to study healthy brain development. In this image of a ferret's brain, neurons (in yellow) are migrating away from their birthplace as the cerebral ...
The importance of animal experiments in brain research. More than 35% of the EU population suffers from brain disorders affecting the lives of individuals as well as their families and loved ones (Raggi and Leonardi, 2020). Most pervasive brain disorders are highly complex, and their etiology often remains poorly understood.
Despite many benefits, the use of animals for research remains a controversial issue. This is also relevant to neuroscience research, in which animals have played an important role for decades. Neuroscience utilizes live animals for two purposes: to understand the functioning of the nervous system and mechanisms of its various pathological states (basic research) and to discover, test and ...
Policymakers aim to move toward animal-free alternatives for scientific research and have introduced very strict regulations for animal research. Homberg et al. argue that, for neuroscience research, until viable and translational alternatives become available and the value of these alternatives has been proven, the use of animals should not be compromised.
We expect that research on the mammalian cerebral cortex will not reveal many new principles—rather it will elaborate the core ones. In general, it should be easier to discover them in simpler brains. Footnote 1. The brain's purpose reduces to regulating the internal milieu—efficiently—and helping the organism to survive and reproduce.
The research comprises behavioural, electrophysiological, molecular, genetic, and neuroimaging studies, with the common aim of contributing to our understanding of the evolution and function of ...
The many ways to map many brains. Ellen P. Neff. Lab Animal 48 , 321-325 ( 2019) Cite this article. 2471 Accesses. 2 Citations. 20 Altmetric. Metrics. Whether the animal has a few hundred ...
Abstract. Policymakers aim to move toward animal-free alternatives for scientific research and have introduced very strict regulations for animal research. We argue that, for neuroscience research, until viable and translational alternatives become available and the value of these alternatives has been proven, the use of animals should not be ...
The brain is directly responsible for governing an animal's interactions with its environment. As such, the brain is often considered to flexibly respond to selection in changing environments (1-3).Brain size is, however, also commonly accepted to be restrained by energetic requirements that are considered universal across all vertebrates (4, 5).
What makes the human brain distinct from that of all other animals — including even our closest primate relatives? In an analysis of cell types in the prefrontal cortex of four primate species, Yale researchers identified species-specific — particularly human-specific — features, they report Aug. 25 in the journal Science.
Animal Brains: An Overview. While they share similar features, no two animal brains are exactly alike. Nicholas Hobbs, a postdoctoral research associate in the neuroscience program at Michigan State University, discusses eight very different animal brains and the unique advantages of each. This video is a clip from the Brain Awareness Week ...
Over the past 30 years, however, research in comparative neuroanatomy clearly has shown that complex brains—and sophisticated cognition—have evolved from simpler brains multiple times ...
Cognition and the Brain; Animal Research; Cite This Article. Weir, K. (2020, January 1). What animal minds can tell us about our own. ... One theme in Rosati's research is studying how and why animals make the decisions they do. The research is inspired, in part, by findings from behavioral economics that show humans make some strange choices
Animal research involving the brain has already produced dramatic improvements in human health and well being, and it promises many more. 18 Drug therapies developed in part in animals have revolutionized the treatment of mental illness in the last generation. For example, chlorpromazine, which was developed in the 1950s using animals, and ...
The vast majority of initial BRAIN Initiative funding was targeted at developing animal models of brain function, and the NIH recently announced a call for applications for creating new colonies of marmosets for "transgenic and chimeric neuroscience research." 5 But despite the rapid acceleration and evolution of neuroscience research on ...
The dearth of treatments for devastating neurological diseases such as Alzheimer's disease and Parkinson's disease is due in large part to the difficulty of conducting research on the human brain, the report says. While research using animal models has advanced understanding, progress is stymied by serious limitations of those models, which ...
Abstract. Animal models are important experimental tools in neuroscience research since they allow appraisal of selected and specific brain pathogenesis-related questions—often not easily accessible in human patients—in a temporal and spatial pattern. Translational research based on valid animal models may aid in alleviating some of the ...
A neuroscientist at the University of Leicester has identified a fundamental difference between human and animal brains. This breakthrough, published today in the journal Cell, offers an explanation for what makes Homo sapiens so vastly different from even our nearest relatives.It could be the key to understanding human intelligence.
Basic neuroscience research in animal models is essential to understanding brain function and the thousands of brain diseases and disorders that affect both humans and animals. Treatments for stroke, depression, and drug addiction are just a few examples of developments made by this research. Learn more.
The new study is an important step in understanding how domestication influences animals' brains, says Erin Hecht, an evolutionary biologist at Harvard University. "Our understanding of brain changes during domestication is still in its infancy," she says. "This study points toward interesting avenues for future brain-behavior research."
Mammals that have evolved more developed brains tend to have a smaller size difference between males and females of that species, according to new research. In many mammal species, the males can ...
In many countries, including the United States and the United Kingdom, research that mixes human brain cells or tissue with another animal's brain is legally allowed, and can be government ...
Scientists have long believed that, generally speaking, the bigger an animal is, the bigger its brain. But our recent study challenges the nature of that linear view and reveals new insights about how brain size and body size have evolved together.. There is astounding diversity in the shape, size and internal structure of the brain among different animals.
Research has long since upended that belief, with studies showing a range of tool use among animals, including orangutans, which create whistles out of leaves to chase away predators; dolphins ...
A multidisciplinary research program. C. elegans is an animal that is simple enough to be studied from the molecular to whole-brain scale, but deceptively complex nonetheless. Though the entire connectome, or wiring diagram, among its 302 neurons has been fully mapped and its genes are readily manipulable in lab experiments, the worm ...
In this study, the researchers analyzed electrical recordings made in the brains of animals that received propofol over an hour-long period, during which they gradually lost consciousness. The recordings were made in four areas of the brain that are involved in vision, sound processing, spatial awareness, and executive function.
Researchers from the University of Kentucky's Sanders-Brown Center on Aging and the University of California Irvine are some of the first to show socialization and enrichment are good for aging brains.. The collaborative study was recently published in the Journal of Neuroscience.Imaging from the work was so compelling it was chosen as the cover art for the issue.
For example, BRAIN aligns with our strategic research goal to improve understanding of the aging brain and support evidence-based strategies to maintain and enhance cognitive, emotional, sensory, and motor function. The BRAIN Initiative is accelerating the development of innovative neurotechnologies to probe brain function spatially and over time.