Information
- Author Services

Initiatives
You are accessing a machine-readable page. In order to be human-readable, please install an RSS reader.
All articles published by MDPI are made immediately available worldwide under an open access license. No special permission is required to reuse all or part of the article published by MDPI, including figures and tables. For articles published under an open access Creative Common CC BY license, any part of the article may be reused without permission provided that the original article is clearly cited. For more information, please refer to https://www.mdpi.com/openaccess .
Feature papers represent the most advanced research with significant potential for high impact in the field. A Feature Paper should be a substantial original Article that involves several techniques or approaches, provides an outlook for future research directions and describes possible research applications.
Feature papers are submitted upon individual invitation or recommendation by the scientific editors and must receive positive feedback from the reviewers.
Editor’s Choice articles are based on recommendations by the scientific editors of MDPI journals from around the world. Editors select a small number of articles recently published in the journal that they believe will be particularly interesting to readers, or important in the respective research area. The aim is to provide a snapshot of some of the most exciting work published in the various research areas of the journal.
Original Submission Date Received: .
- Active Journals
- Find a Journal
- Proceedings Series
- For Authors
- For Reviewers
- For Editors
- For Librarians
- For Publishers
- For Societies
- For Conference Organizers
- Open Access Policy
- Institutional Open Access Program
- Special Issues Guidelines
- Editorial Process
- Research and Publication Ethics
- Article Processing Charges
- Testimonials
- Preprints.org
- SciProfiles
- Encyclopedia
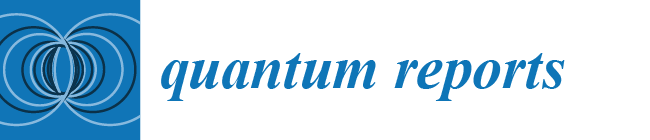
Journal Menu
- Quantum Reports Home
- Aims & Scope
- Editorial Board
- Instructions for Authors
- Special Issues
- Article Processing Charge
- Indexing & Archiving
- Most Cited & Viewed
- Journal Statistics
- Journal History
- Journal Awards
- Conferences
- Editorial Office
Journal Browser
- arrow_forward_ios Forthcoming issue arrow_forward_ios Current issue
- Vol. 6 (2024)
- Vol. 5 (2023)
- Vol. 4 (2022)
- Vol. 3 (2021)
- Vol. 2 (2020)
- Vol. 1 (2019)
Find support for a specific problem in the support section of our website.
Please let us know what you think of our products and services.
Visit our dedicated information section to learn more about MDPI.
Recent Advances in Quantum Biology
- Print Special Issue Flyer
- Special Issue Editors
Special Issue Information
Benefits of publishing in a special issue.
- Published Papers
A special issue of Quantum Reports (ISSN 2624-960X).
Deadline for manuscript submissions: closed (31 December 2021) | Viewed by 47708
Share This Special Issue
Special issue editor.

Dear Colleagues,
One of the great challenges of modern science is to bridge the gap between atomic and cellular level phenomena that affect outcomes in living systems. A potentially transformational facet of this challenge is quantum biology: understanding how quantum properties play governing roles in biological functions. For example, key mechanisms for bird navigation, olfactory sensing, and photosynthesis implicate quantum effects in biological systems. The defining feature of quantum biology is that quantum effects such as coherence and superposition are found at room temperature, in wet environments that typically have lots of motion. Implementation of these principles can lead to a new generation of bio-inspired quantum technologies that can function at ambient temperature and will change the way we think about our world, with applications for improved regenerative medicine, enhanced wound healing, improved human performance, efficient solar energy harvesting, and vision based magnetoreception.
The present Special Issue “Recent Advances in Quantum Biology” aims to collect and publish recent advances in the area of quantum biology. We welcome all reviews and research articles concerned with molecular level quantum phenomena observed in biological systems at functional, cellular, or organism levels. This includes also the disruptive impact of emerging areas of quantum biology. Topics with special emphasis are summarized in the keywords below.
Dr. Carlos F. Martino Guest Editor
Manuscripts should be submitted online at www.mdpi.com by registering and logging in to this website . Once you are registered, click here to go to the submission form . Manuscripts can be submitted until the deadline. All submissions that pass pre-check are peer-reviewed. Accepted papers will be published continuously in the journal (as soon as accepted) and will be listed together on the special issue website. Research articles, review articles as well as short communications are invited. For planned papers, a title and short abstract (about 100 words) can be sent to the Editorial Office for announcement on this website.
Submitted manuscripts should not have been published previously, nor be under consideration for publication elsewhere (except conference proceedings papers). All manuscripts are thoroughly refereed through a single-blind peer-review process. A guide for authors and other relevant information for submission of manuscripts is available on the Instructions for Authors page. Quantum Reports is an international peer-reviewed open access quarterly journal published by MDPI.
Please visit the Instructions for Authors page before submitting a manuscript. The Article Processing Charge (APC) for publication in this open access journal is 1400 CHF (Swiss Francs). Submitted papers should be well formatted and use good English. Authors may use MDPI's English editing service prior to publication or during author revisions.
- photosynthesis
- magnetoreception
- radical pair formation in cryptochromes
- reactive oxygen species production
- ATP production
- Ease of navigation: Grouping papers by topic helps scholars navigate broad scope journals more efficiently.
- Greater discoverability: Special Issues support the reach and impact of scientific research. Articles in Special Issues are more discoverable and cited more frequently.
- Expansion of research network: Special Issues facilitate connections among authors, fostering scientific collaborations.
- External promotion: Articles in Special Issues are often promoted through the journal's social media, increasing their visibility.
- e-Book format: Special Issues with more than 10 articles can be published as dedicated e-books, ensuring wide and rapid dissemination.
Further information on MDPI's Special Issue polices can be found here .
Published Papers (7 papers)
Jump to: Review
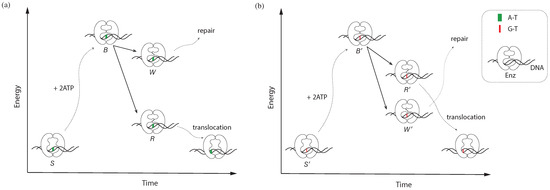
Jump to: Research
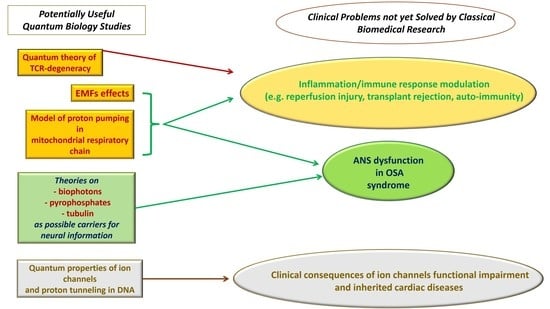
Graphical abstract
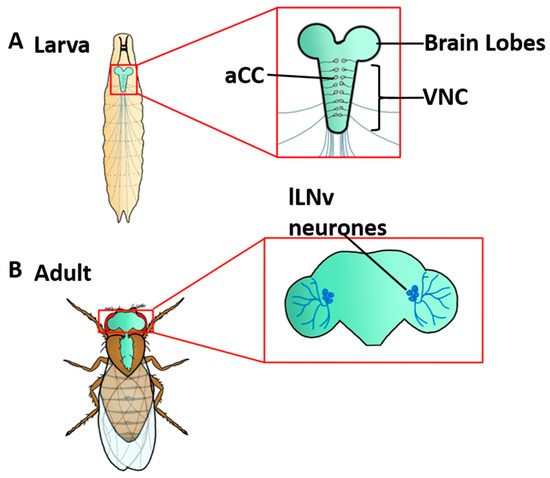
Further Information
Mdpi initiatives, follow mdpi.
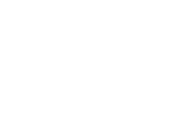
Subscribe to receive issue release notifications and newsletters from MDPI journals
Grab your spot at the free arXiv Accessibility Forum
Help | Advanced Search
Quantitative Biology > Other Quantitative Biology
Title: quantum biology at the cellular level - elements of the research program.
Abstract: Quantum Biology is emerging as a new field at the intersection between fundamental physics and biology, promising novel insights into the nature and origin of biological order. We discuss several elements of QBCL (Quantum Biology at Cellular Level), a research program designed to extend the reach of quantum concepts to higher than molecular levels of biological organization. Key words. decoherence, macroscopic superpositions, basis-dependence, formal superposition, non-classical correlations, Basis-Dependent Selection (BDS), synthetic biology, evolvability mechanism loophole.
Comments: | 53 pages, 8 figures, with 3 open reviews |
Subjects: | Other Quantitative Biology (q-bio.OT) |
Cite as: | [q-bio.OT] |
(or [q-bio.OT] for this version) | |
Focus to learn more arXiv-issued DOI via DataCite |
Submission history
Access paper:.
- Other Formats
References & Citations
- Google Scholar
- Semantic Scholar
BibTeX formatted citation

Bibliographic and Citation Tools
Code, data and media associated with this article, recommenders and search tools.
- Institution
arXivLabs: experimental projects with community collaborators
arXivLabs is a framework that allows collaborators to develop and share new arXiv features directly on our website.
Both individuals and organizations that work with arXivLabs have embraced and accepted our values of openness, community, excellence, and user data privacy. arXiv is committed to these values and only works with partners that adhere to them.
Have an idea for a project that will add value for arXiv's community? Learn more about arXivLabs .
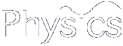
- Collections
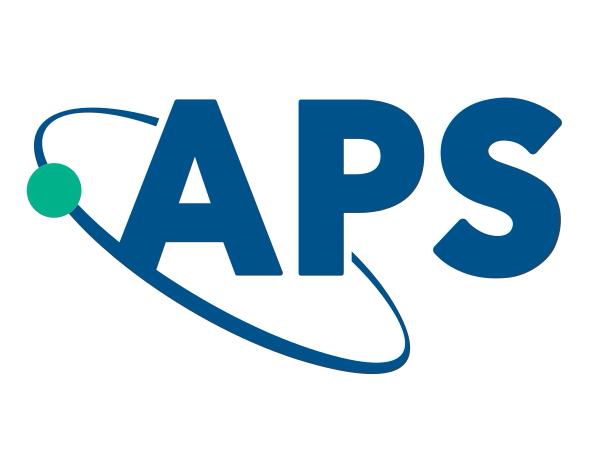
- APS Journals
It’s Time to Take Quantum Biology Research Seriously
- Samueli School of Engineering, University of California, Los Angeles, Los Angeles, CA, US
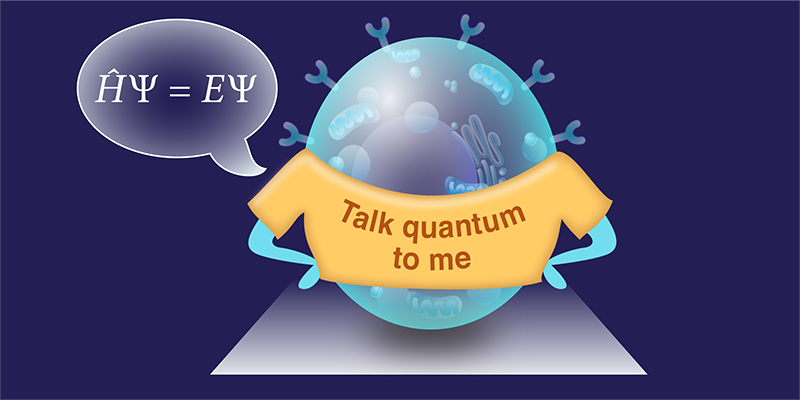
Imagine healing an injury by applying a tailored magnetic field to a wound. This outcome might sound fantastical, but researchers have shown that cell proliferation and wound healing, among other important biological functions, can be controlled by magnetic fields with strengths on the order of those produced by cell phones. This kind of physiological response is consistent with one caused by quantum effects in electron spin-dependent chemical reactions. However (and it’s a big however), while researchers have unambiguously established such reactions for in vitro experiments, they have not done so for in vivo studies. The barriers to in vivo experiments stem both from the absence of experimental infrastructure to perform true quantum measurements inside biological systems and from a misunderstanding of what quantum behaviors in biology are and why they matter. In my opinion, it is time to set the record straight so that we can legitimize work in this field. Quantum biology findings could enable the development of new drugs and of noninvasive therapeutic devices to heal the human body, as well as provide an opportunity to learn how nature builds its own quantum technologies.
Quantum biology researchers study the inherent quantum degrees of freedom of biological matter with the goal of understanding and controlling these phenomena. To a physicist, I’d describe quantum biology as the study of light–matter interactions, where the matter is living. Quantum biology is not the study of classical biology using quantum tools, nor is it the application of quantum computers or of quantum machine learning to drug discovery or healthcare big data processing, and it definitely has nothing to do with the manipulation of free will, with the origin of consciousness, or with other New Age buzzwords.
Experimental evidence consistent with quantum effects existing in biological systems has been around for more than 50 years. One example is the spin-dependent chemical reaction thought to allow birds to navigate using Earth’s weak magnetic field. Today, there is no doubt that such phenomena play important roles in laboratory biological systems—for example, it is uncontroversial that quantum superpositions can manifest in proteins in solution for long enough that they influence chemical processes. But as yet there is no unambiguous experimental evidence that a single living cell can maintain or utilize quantum superposition states within its molecules, as is required, for example, if birds truly use a quantum process as a compass.
This lack of experimental verification is one of the main reasons that the field is considered inconsequential by funders and by the established quantum and biophysics communities. Yes, sophisticated experiments have been performed with single molecules in solution and with whole organisms (birds and flies, for example). But these experiments only show correlation, not causation, between a molecule’s or an organism’s behavior and quantum physics. Bridging that gap will require performing truly quantum measurements inside biological matter using challenging combinations of quantum instrumentation and wet lab techniques.
Another reason quantum biology is not considered a legitimate field of science is the absence of a cohesive quantum biology community. That deficit is beginning to change, but further efforts are needed in that direction. In early 2020, people in my lab and in the Quantum Biology Doctoral Training Centre at the University of Surrey, UK, started an online seminar series called Big Quantum Biology Meetings. The seminars provide a forum for the more than 600 quantum biology researchers and enthusiasts signed up to our mailing list to meet informally once a week. Other efforts to create a cohesive community include establishing a Gordon Research Conference on Quantum Biology, the first of which happened earlier this year and was attended by 150 people, and the gaining of support from the National Science Foundation for a Research Coordination Network on “Instrumentation for Quantum Biology.”
A point of pride of the Big Quantum Biology Meetings series is the intentional incorporation of inclusive practices in the seminars. For example, each meeting starts with a short presentation from a trainee, which we define as anyone without a permanent position, giving them and their work exposure. The trainee is then the host and mediator for the rest of the meeting. The main speaker also gives a “DEIJ moment”—one slide on anything related to diversity, equity, inclusion, and justice that has impacted their scientific life.
A final reason why quantum biology struggles in being accepted as a stand-alone field is the continued presence of scientific silos at institutions. If cells and organisms are using quantum effects to function optimally, a cohort of interdisciplinary experts is needed to collaboratively explore the problem. In my opinion, this collaboration would ideally take place in a quantum biology-focused institute where scientists can easily and organically work together. Recently, in an example of this idea, Japan unveiled the Institute for Quantum Life Science, which brings chemists, biologists, engineers, clinicians, physicists, and others under one roof to work on quantum biology research questions. The development of a similar institute in the US could help in irrevocably establishing this field—which will have, I believe, radical consequences for the biological, medical, and physical sciences.
About the Author
Clarice Aiello is a quantum engineer interested in how quantum physics informs biology at the nanoscale. She is an expert on nanosensors that harness room-temperature quantum effects in noisy environments. Aiello received a bachelor’s in physics from the Ecole Polytechnique, France; a master’s degree in physics from the University of Cambridge, Trinity College, UK; and a PhD in electrical engineering from the Massachusetts Institute of Technology. She held postdoctoral appointments in bioengineering at Stanford University and in chemistry at the University of California, Berkeley. Two months before the pandemic, she joined the University of California, Los Angeles, where she leads the Quantum Biology Tech (QuBiT) Lab.
Recent Articles
How to Detect a Stream of Microwave Photons
A new device converts a stream of microwave photons into an electric current with high efficiency, which will benefit quantum information technologies. Read More »
Setting Temporal Boundaries for Sound Waves
A magnet-and-coil system reveals how acoustic waves reflect and refract when the host medium suddenly changes elasticity. Read More »
Mapping the Textures of Thicker Magnets
A soft x-ray magnetic imaging technique makes possible the study of a wide range of magnetic materials. Read More »
Sign up to receive weekly email alerts from Physics Magazine .
Academia.edu no longer supports Internet Explorer.
To browse Academia.edu and the wider internet faster and more securely, please take a few seconds to upgrade your browser .
- We're Hiring!
- Help Center
Quantum Biology
- Most Cited Papers
- Most Downloaded Papers
- Newest Papers
- Last »
- Non-equilibrium thermodynamics Follow Following
- Quantum Coherence Follow Following
- Epigenetics Follow Following
- Quantum Chaos Follow Following
- Network science Follow Following
- Statistical Physics Of Complex Systems Follow Following
- Smart Cities Follow Following
- Water Follow Following
- Quantum Electrodynamics Follow Following
- Bioelectromagnetics Follow Following
Enter the email address you signed up with and we'll email you a reset link.
- Academia.edu Journals
- We're Hiring!
- Help Center
- Find new research papers in:
- Health Sciences
- Earth Sciences
- Cognitive Science
- Mathematics
- Computer Science
- Academia ©2024
Local Navigation
Site Navigation
General Lab Information

Studying Loss to Make Quantum Computing Gains
By characterizing sources of energy loss and optimizing quantum circuits, scientists have reached a major performance milestone for quantum devices.
August 12, 2024
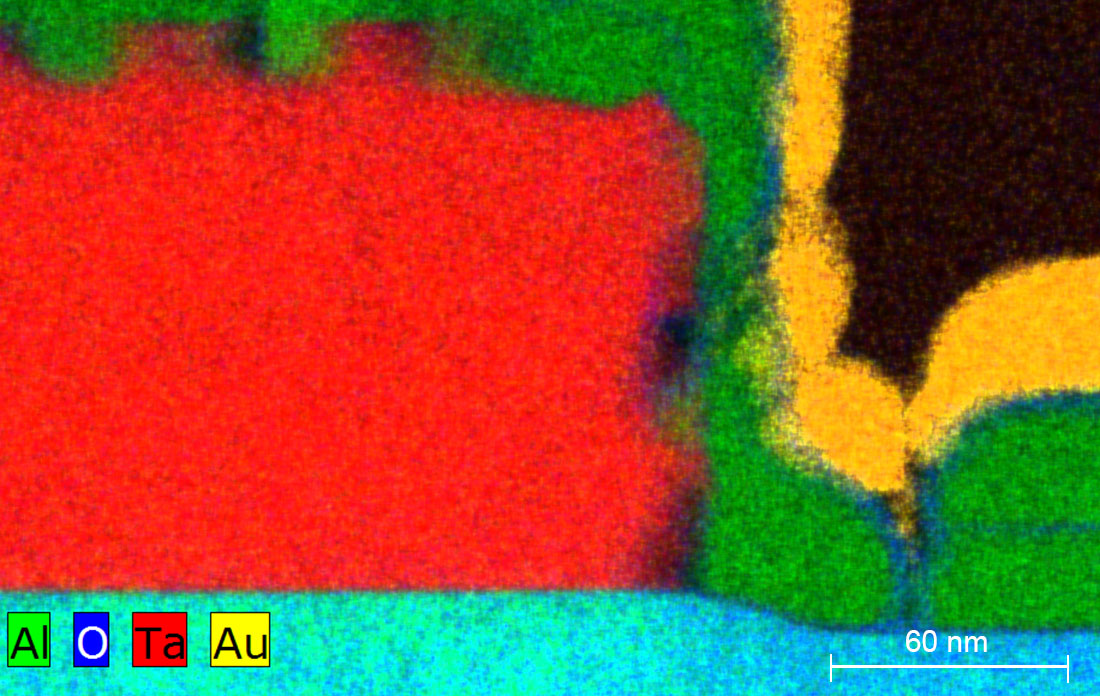
Scientists at the Center for Functional Nanomaterials used transmission electron microscopy to analyze the elemental makeup of materials comprising quantum devices. The above image shows that there is no oxide between the tantalum (Ta) and aluminum (Al) layers. This indicates that there is good metal-to-metal contact, which is important for quantum devices exhibiting high coherence. (Brookhaven National Laboratory)
UPTON, N.Y. — Scientists from Yale University and the U.S. Department of Energy’s (DOE) Brookhaven National Laboratory have developed a systematic approach to understanding how energy is lost from the materials that make up qubits. Energy loss inhibits the performance of these quantum computer building blocks, so determining its sources — and adjusting the materials as necessary — can help bring researchers closer to designing quantum computers that could revolutionize several scientific fields. With their new approach, the Yale scientists were able to design a compact device that could store quantum information for more than one millisecond.
This research, recently published in Nature Communications , was conducted as part of the Co-Design Center for Quantum Advantage (C 2 QA), a DOE-funded national quantum information science research center led by Brookhaven Lab. Yale is a key partner of the center.
“A significant hurdle that we must overcome is improving the ability of qubits to retain the quantum information encoded in them. This is known as coherence,” explained Suhas Ganjam, who is first author on the new paper. Ganjam conducted the research as a doctoral student at Yale and is now a research scientist at Google.
A few years ago, Princeton University researchers — who joined C 2 QA upon its establishment — designed qubits with a record-breaking coherence time of 0.3 milliseconds by replacing the traditionally used niobium or aluminum with a superconducting metal called tantalum. This indicated that qubits’ constituent materials directly affect their performance, but the reasons for this were still unclear.
So, scientists contributing to C 2 QA began investigating the different kinds of tantalum oxides that form on tantalum’s surface when it is exposed to air. They further improved coherence by coating tantalum with a thin layer of magnesium that prevented the material’s oxidation.
“Researchers have been building devices with better coherence times. But there are so many different sources of energy loss, and we still couldn’t distinguish which ones were improving,” said Ganjam. “So, we set out to differentiate between the different types of loss.”
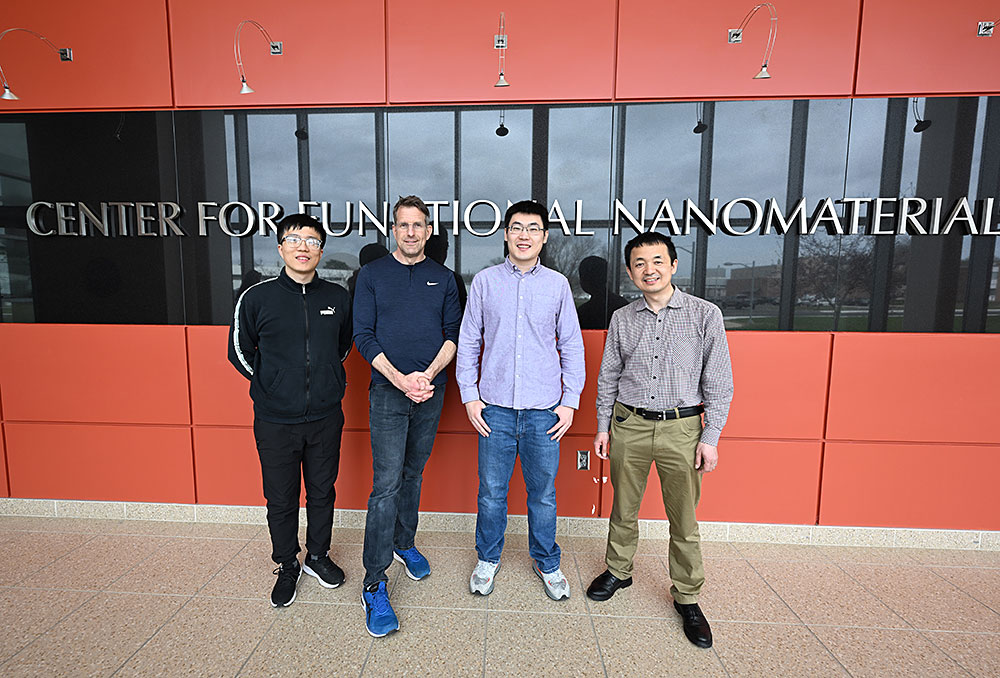
Brookhaven researchers Chenyu Zhou, Kim Kisslinger, Ruoshui Li, and Mingzhao Liu (left to right) leveraged advanced materials characterization techniques at the Center for Functional Nanomaterials to figure out how certain materials and fabrication techniques affect the performance of quantum devices. (Kevin Coughlin/Brookhaven National Laboratory)
Under the supervision of Robert Schoelkopf, a physicist at Yale University who leads the Devices Thrust of C 2 QA, Ganjam designed a device called a tripole stripline. This new device consists of three superconducting thin-film strips patterned on a substrate, similar to other quantum devices. The strips were arranged in a special way so that the researchers could not only quantify energy lost but also determine where it was being lost by testing the device in three different modes — one for each pair of superconducting electrodes.
For example, the researchers could differentiate between surface loss and bulk dielectric loss by observing modes in which electromagnetic fields were either confined to the surface of the device or spread throughout the substrate. If they observed more loss from the mode in which electromagnetic fields were confined to the surface of the device, the loss was dominated by the surface contribution.
“Through our electromagnetic tests with the tripole stripline, we could observe that devices made with tantalum and aluminum lose different amounts of energy in different ways,” Ganjam explained.
In particular, the researchers found that using a tantalum thin film, rather than an aluminum thin film, reduced surface loss. And using a fabrication technique called annealing, which involves heating a sapphire substrate and letting it cool slowly, reduced the bulk dielectric loss.
“We wanted to know why the different materials and fabrication techniques influenced loss like this,” Ganjam said. “So, we turned to our collaborators from the Center for Functional Nanomaterials.”
Quantum materials through the microscopy lens
The Center for Functional Nanomaterials (CFN) is a DOE Office of Science user facility at Brookhaven Lab with a state-of-the-art Electron Microscopy facility. Using transmission electron microscopy and scanning transmission electron microscopy to look at the materials’ microscopic structure, scientists from this facility can help other researchers, like Ganjam and Schoelkopf, better understand the materials they are working with.
“We suspect that qubit coherence is limited by energy loss that is due to contaminants or defects in the materials,” explained Minghzao Liu, a senior scientist at CFN. “So, we analyze the quantum materials at CFN to look for these coherence-limiting characteristics.”
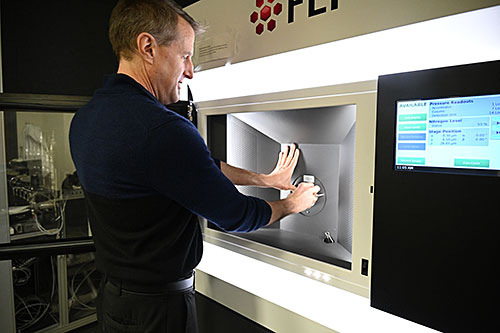
Kim Kisslinger, an advanced technical associate at the Center for Functional Nanomaterials, used transmission electron microscopy to examine microscopic cross-sections of quantum materials and devices. (Kevin Coughlin/Brookhaven National Laboratory)
Kim Kisslinger, an advanced technical associate at CFN, extracted microscopic cross-sections of the Yale scientists’ materials and devices and analyzed them at the atomic level.
“I view projects like this through an electron microscopy lens,” said Kisslinger. “From crystallinity to chemical composition to epitaxy, which is related to the orientation of the crystal materials, I can tell our collaborators exactly what is going on with their materials and help them correlate these properties with the materials’ performance.”
Liu said, “Kim helps our collaborators better understand their materials, but he also helps them make meaningful improvements through an iterative process.”
Kisslinger added, “CFN is home to cutting-edge equipment that can support the materials research needed for quantum devices. But we also have some of the most qualified scientists and specialists in the world. This combination of quality people and quality equipment is unique to CFN.”
Collaborative efforts yield improved devices
With a well-rounded understanding of the electromagnetic properties of their devices, as well as the material composition, the Yale researchers utilized an energy loss model that could predict a device’s coherence based on its constituent materials and the circuit geometry. And with the help of this predictive model, they optimized circuit geometry to build a quantum device with a coherence time greater than one millisecond.
“CFN is home to cutting-edge equipment that can support the materials research needed for quantum devices. But we also have some of the most qualified scientists and specialists in the world.”
— Kim Kisslinger, advanced technical associate at CFN
“This research marks an important milestone in the C 2 QA mission. Even beyond the longer coherence time, it demonstrates a path forward to further coherence enhancements through the close collaboration of quantum device and materials scientists,” said C 2 QA Deputy Director Kai-Mei Fu.
The collaboration between the qubit design experts from the Schoelkopf lab and CFN materials characterization experts, which began with the establishment of the center, embodies C 2 QA’s principle of “co-designing” materials and algorithms to achieve quantum computers that outperform classical computers.
“Collaborations like this one are key to unlocking the best materials and optimal fabrication processes that will help C 2 QA realize their goal,” Ganjam said.
“It has been quite rewarding to see these qubit design projects grow in scope and success over the years,” added Liu. “Scientific advances like this are not possible without collaboration.”
Brookhaven National Laboratory is supported by the Office of Science of the U.S. Department of Energy. The Office of Science is the single largest supporter of basic research in the physical sciences in the United States and is working to address some of the most pressing challenges of our time. For more information, visit science.energy.gov .
Follow @BrookhavenLab on social media. Find us on Instagram , LinkedIn , X , and Facebook .
Related Links
- Scientific paper: Surpassing millisecond coherence in on chip superconducting quantum memories by optimizing materials and circuit design
- Understanding the Tantalizing Benefits of Tantalum for Improved Quantum Processors
2024-21930 | INT/EXT | Newsroom
Other Articles...
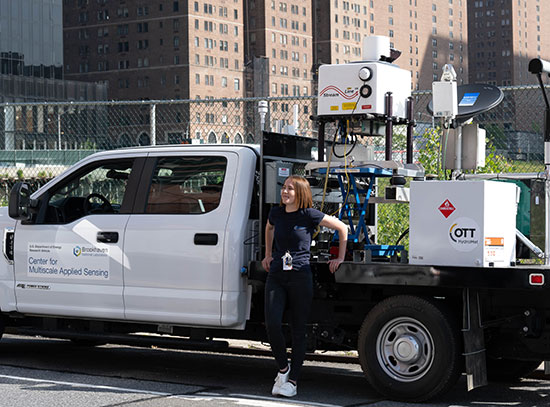
DOE Announces $10 Million to Support Climate Resilience Centers Across America
Friday, August 16, 2024
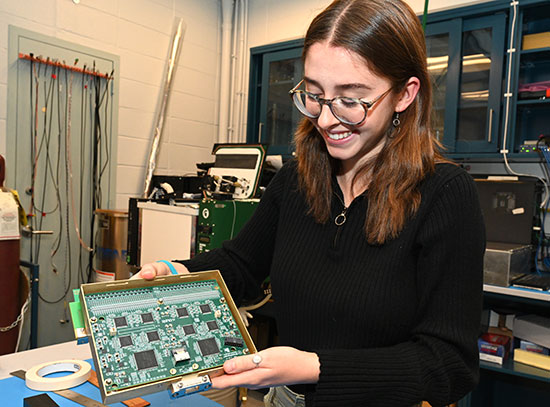
The More You Neutrino…
Thursday, August 15, 2024
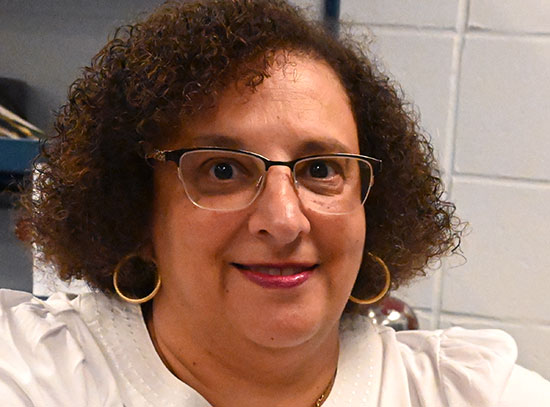
Mary Bishai Named Distinguished Scientist Fellow
Monday, August 12, 2024
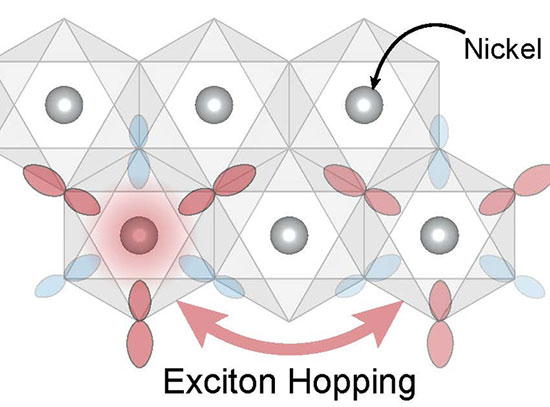
Physicists Report New Insights Into Exotic Particles Key to Magnetism
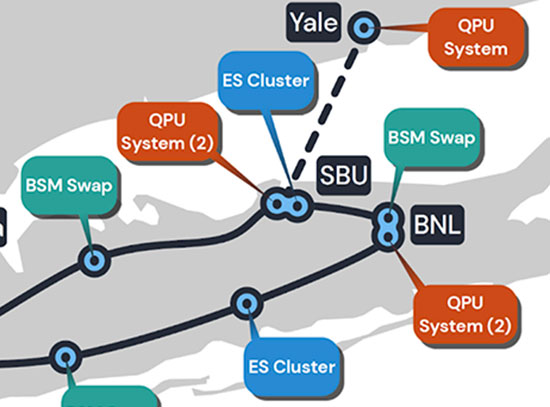
SBU Collaboration to Lead Project in National Quantum Virtual Laboratory Program
Friday, August 9, 2024
Brookhaven National Laboratory
Brookhaven National Laboratory is a multipurpose research institution funded by the U.S. Department of Energy. Located on Long Island, NY, Brookhaven operates large-scale facilities for studies in physics, chemistry, biology, medicine, applied science, and advanced technology. The Laboratory's almost 3,000 scientists, engineers, and support staff are joined each year by more than 5,000 visiting researchers from around the world.
PO Box 5000 Upton, NY 11973-5000 (631) 344-8000
- Our Science
- Visiting the Lab
- Staff Directory
- Guest Center
- Partnerships
- For Vendors
- Departments
- Public Events
- Diversity, Equity & Inclusion
- Technology Licensing
- Stakeholder Relations
- Students & Educators
- Sustainability
- Privacy and Security Notice
- Vulnerability Disclosure Program
Brookhaven Science Associates
Brookhaven Science Associates manages and operates Brookhaven National Laboratory on behalf of the U.S. Department of Energy's Office of Science. BSA is a partnership between Battelle and The Research Foundation for the State University of New York on behalf of Stony Brook University. | More

Thank you for visiting nature.com. You are using a browser version with limited support for CSS. To obtain the best experience, we recommend you use a more up to date browser (or turn off compatibility mode in Internet Explorer). In the meantime, to ensure continued support, we are displaying the site without styles and JavaScript.
- View all journals
- Explore content
- About the journal
- Publish with us
- Sign up for alerts
- Review Article
- Published: 03 February 2023
Quantum sensors for biomedical applications
- Nabeel Aslam 1 , 2 , 3 ,
- Hengyun Zhou 1 ,
- Elana K. Urbach 1 ,
- Matthew J. Turner 4 , 5 ,
- Ronald L. Walsworth 4 , 5 , 6 ,
- Mikhail D. Lukin 1 &
- Hongkun Park ORCID: orcid.org/0000-0001-9576-8829 1 , 2
Nature Reviews Physics volume 5 , pages 157–169 ( 2023 ) Cite this article
46k Accesses
97 Citations
177 Altmetric
Metrics details
- Confocal microscopy
- Imaging and sensing
- Nanosensors
- Quantum metrology
- Solution-state NMR
Quantum sensors are finding their way from laboratories to the real world, as witnessed by the increasing number of start-ups in this field. The atomic length scale of quantum sensors and their coherence properties enable unprecedented spatial resolution and sensitivity. Biomedical applications could benefit from these quantum technologies, but it is often difficult to evaluate the potential impact of the techniques. This Review sheds light on these questions, presenting the status of quantum sensing applications and discussing their path towards commercialization. The focus is on two promising quantum sensing platforms: optically pumped atomic magnetometers, and nitrogen–vacancy centres in diamond. The broad spectrum of biomedical applications is highlighted by four case studies ranging from brain imaging to single-cell spectroscopy.
Quantum sensors can detect magnetic fields and other physical quantities, with unprecedented spatial resolution and sensitivity, making them highly interesting for biomedical applications.
Optically pumped magnetometers offer new functionalities in clinical magnetoencephalography, with their wearable sensor helmet allowing the subject to perform tasks and move during the recording of brain activity.
Nitrogen–vacancy (NV)-centre-based magnetometry of single neurons and magnetic biomarkers, with subcellular resolution, opens new avenues in studying neuronal circuits and in rapid clinical testing.
Nuclear magnetic resonance based on NV centres in diamond enables microscale and nanoscale detection of single molecules and single cells, which could be applied in structure determination of transmembrane proteins and in metabolomics studies.
Nanodiamonds containing NV centres can locally probe temperature-dependent biological processes in cells and small organisms, such as cell development and endogenous heat generation.
Similar content being viewed by others
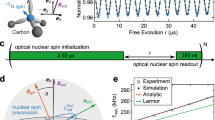
All-optical nuclear quantum sensing using nitrogen-vacancy centers in diamond

Correlated sensing with a solid-state quantum multisensor system for atomic-scale structural analysis
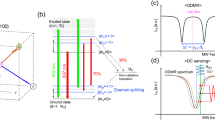
Magnetometer with nitrogen-vacancy center in a bulk diamond for detecting magnetic nanoparticles in biomedical applications
Introduction.
Advances in biomedical sciences are often spurred by the development of tools with enhanced sensitivity and resolution, which allow detection and imaging of signals that are progressively weaker, more localized and/or biologically specific. Improvements in nuclear magnetic resonance (NMR) or magnetoencephalography (MEG) have resulted in tremendous progress in diagnostics and treatment, yet further progress in sensitivity and resolution seems to be challenging with conventional methods. However, a promising direction for a new generation of biomedical sensors with greatly enhanced performance comes from advances in quantum science and technology.
Control and measurement of individual quantum systems, which seemed impossible only a few decades ago, is now a reality in many laboratories worldwide. This achievement has generated much excitement in both academia and industry. Quantum computation and quantum communication have garnered great attention 1 , 2 , 3 , but quantum sensing, another pillar of quantum technology, is also advancing rapidly. One advantage of quantum sensors is the improvement in sensitivity that stems from quantum effects. Some quantum sensors are as small as a single atom and can consequently yield unmatched spatial resolution. These new capabilities could lead to a true ‘quantum leap’ in biomedical applications. First attempts have already been made, in the form of quantum-sensor-based brain imaging 4 and NMR at the scale of individual proteins and cells 5 , 6 . This is an exciting time for quantum sensing as it transitions from academic laboratories to commercial applications.
The focus of this Review is on two promising quantum platforms as exemplars of quantum sensors in biomedical applications: optically pumped magnetometers (OPMs) and magnetometry based on nitrogen–vacancy (NV) centres in diamond. An overview of the potential applications on the molecular, cellular and organism levels is given in Fig. 1 . We present four case studies, chosen among the many directions that are being actively explored. The first is MEG based on OPMs, with an emphasis on its advantages over conventional MEG based on superconducting quantum interference devices (SQUIDs). The second case study discusses how diamond sensor chips with an ensemble of NV centres can be used for magnetic sensing and microscopy of biological cells and tissue. The third is dedicated to NV-diamond sensing applied to nanoscale and microscale NMR imaging and spectroscopy. The final case study will discuss nanoscale thermometry with NV centres in nanodiamonds. For discussion of the principles of quantum sensing, we refer readers to other reviews 7 , 8 , 9 , 10 .
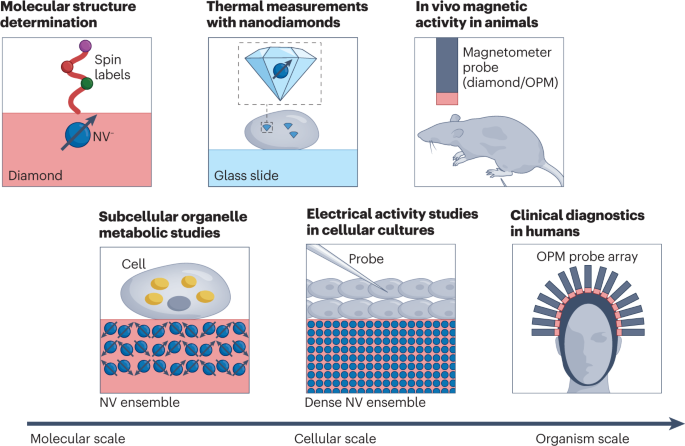
Nitrogen–vacancy (NV) centres in diamond are suited for structure determination of single molecules. On the cellular scale, NV centres could help study metabolism and probe electrical activity of neurons. Such quantum sensors can also be integrated into nanodiamonds and serve as in vivo nanoscale temperature sensors. Detection of biomagnetic signal from animals and humans is another promising application of quantum sensors. In this regard, optically pumped magnetometers (OPM) are well suited due to their high magnetic field sensitivity.
What are quantum sensors?
Quantum sensors are individual systems or ensembles of systems that use quantum coherence, interference and entanglement to determine physical quantities of interest 7 . In what follows, we do not limit the discussion to entanglement-enhanced sensing, although there have been numerous early demonstrations of such capabilities in the field.
Quantum sensors have been realized in multiple systems with very different operating principles. This diversity makes them each suitable for different applications and allows them to be used in complementary ways. In the following, we give a brief overview of the most prominent examples of quantum sensors.
Superconducting circuits
One of the earliest quantum sensors is the SQUID. Based on superconducting Josephson junctions, SQUIDs can measure magnetic fields with sensitivities reaching 10 aT Hz −1/2 in controlled laboratory settings 11 . Many SQUID sensors are commercially available and are, for example, applied in MEG, where they detect magnetic signals from the brain. However, SQUIDs require cryogenic operation, which necessitates bulky set-ups and limits the achievable spatial resolution for real-world applications.
Atomic ensembles
Alkali atom ensembles in vapour cells can be spin-polarized, and their magnetic field coupling can be probed through optical means (Fig. 2a ). Besides the absorption-based approach described in Fig. 2a , magnetic field detection can also be achieved by measuring d.c. and a.c. polarization rotation of the probe light 8 . These OPMs can achieve minute-long coherence times 12 and magnetic field sensitivities of 100 aT Hz −1/2 in the laboratory 13 . Spin-exchange relaxation-free OPMs are operated at elevated temperatures of 100 °C and near-zero magnetic field. The high temperature requires insulation from target samples, and the requirement for near-zero magnetic field necessitates additional coils to cancel out environmental magnetic fields. OPM cells have been miniaturized down to the millimetre scale, but with degradation in sensitivity, ultimately limiting the spatial resolution for a given sensitivity 14 . Entanglement-enhanced sensing has also been demonstrated with OPMs 15 , 16 . The combination of these properties has enabled OPM application in NMR 17 , MEG 4 , magnetocardiography (MCG) 18 and magnetic induction tomography 19 , 20 .
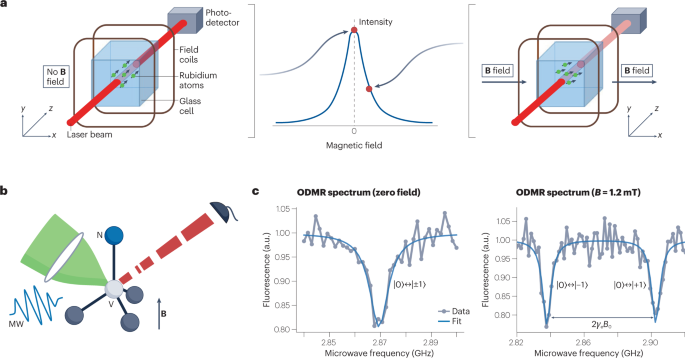
a , In an OPM, rubidium atoms (as an example out of the various alkali atoms being used) in a glass cell are optically spin-polarized. In zero magnetic field, the transmission of a probe laser is at its maximum (left). The presence of a magnetic field leads to Larmor precession of the spins, which reduces transmission of the probe laser (right). b , The NV centre in the diamond lattice has its spin state initialized and interrogated via green excitation light, microwave (MW) resonant fields and red fluorescence, in the presence of magnetic fields ( B ). c , Optically detected magnetic resonance (ODMR) spectrum of the NV centre at zero field (left) and at B = 1.2 mT (right), where γ is the electron gyromagnetic ratio and |0 ⟩ , |±1 ⟩ stand for the electron spin states m s = 0, ±1, respectively. Part a adapted with permission from ref. 4 , Springer Nature Ltd.
Solid-state spins
Electronic spin defects in semiconductors can also be used for quantum sensing. Diamond stands out as a special host material for optically addressable defects, owing to its large bandgap. Most notably, the NV colour centre in diamond has been widely studied as a quantum sensor 21 , 22 . Optical initialization and readout of the spin state has been demonstrated down to the single-NV level 23 (Fig. 2b ). Owing to low spin–orbit coupling, the NV’s ground-state spin can reach coherence times of ~1 ms under ambient conditions 24 . NV centres have been shown to function over large ranges of temperature (4 K to 625 K) 25 , magnetic field (up to 8.3 T) 26 and pressure (up to 13.6 GPa) 27 . These properties make the NV and other colour centres in diamond 28 attractive not only for quantum sensing but also for quantum information processing 29 and quantum communication 3 . For magnetometry, the photon shot-noise-limited sensitivity can be enhanced by a factor of 1/ \(\sqrt{N}\) by using an ensemble of N NV centres, with an experimentally achieved sensitivity of ~1 pT Hz −1/2 (ref. 30 ). In addition, NV centres can be used as a sensor for temperature 31 , electric fields 32 and pressure 27 .
NV centres exhibit a sensing bandwidth ranging from d.c. to gigahertz frequencies 33 . In wide-field imaging applications using NV ensembles, the spatial resolution is typically set by the diffraction limit of the optical microscope. However, by combining NVs with scanning techniques, such as atomic force microscopy, the spatial resolution can be improved to the few-nanometre level, mainly limited by the NV-to-surface distance 34 . It is also possible to create nanodiamonds containing NV centres, which can be functionalized and serve as local probes 31 . Other defects in diamond (such as silicon vacancy centres 28 ) and defects in different host materials (such as silicon carbide 35 or yttrium orthosilicate 36 ) are being actively investigated as quantum sensors. As yet, they do not show sensitivities comparable to the NV centres, owing to their limited coherence properties.
In this Review, we highlight biomedical applications of OPMs and diamond NV centres as an illustration of the broad operating regimes that quantum sensors can cover. The OPMs and NV centres have complementary strengths and weaknesses. The high sensitivity of OPMs makes them suitable for macroscopic detection of weak magnetic fields, such as those generated by the brain or the heart. Conversely, a major advantage of NV centres is the short sensor-to-sample distance, allowing high spatial resolution and high sensitivity to weak, microscopic signals. In addition, the NV centre is a multifunctional sensor (detecting a.c. and d.c. magnetic fields, temperature and so on) and operates under a wide range of conditions. This multifunctionality makes it attractive for spectroscopy and diagnostics on the cellular level.
OPM-based MEG
Monitoring and imaging biomagnetism from the human body is useful for diagnostic and treatment purposes 37 . For example, the brain produces magnetic fields through the flow of electric currents in neurons. These fields are detected by MEG and can be used to study brain injury 38 and brain disorders such as epilepsy 39 and dementia 40 .
Conventional MEG, as first demonstrated in the early 1970s, is based on SQUID sensors 41 , 42 and exhibits a noise level of fT Hz −1/2 . Despite considerable commercial and clinical adoption, the demanding operation conditions of MEG still pose severe limitations. For example, SQUID operation requires cryogenic temperatures, which makes the sensor array bulky (weighing over 400 kg for the helmet that holds the sensor array) and increases the sensor-to-subject distance. This distance not only limits signal-to-noise ratio and spatial resolution but also makes it infeasible for the subject to move during the recording. Moreover, the fixed MEG helmet’s size complicates brain imaging because the subject’s head profile can vary significantly, especially in the case of children.
The emergence of quantum sensing techniques has opened new avenues to tackle these limitations. Most promising are commercially available OPMs that can achieve sensitivities of ~10 fT Hz −1/2 (ref. 43 ), comparable to clinical SQUID devices. OPMs do not require low temperatures, thus simplifying the sensor architecture and allowing short sensor-to-sample distances. Another benefit of OPMs is their ability to detect vector magnetic fields 44 , 45 , 46 , whereas SQUIDs only measure the magnetic field component radial to the scalp surface. Such triaxial detection results in an overall higher signal strength and helps differentiate signal and background fields. Furthermore, progress in miniaturization of OPMs has enabled prototype OPM-MEGs to be built 4 (Fig. 3a ), paving the way towards real-world applications.

a , Prototype wearable OPM-MEG. The subject can move their head during the measurement, as exemplified by the subject bouncing a tennis ball off a bat. b , Beta band oscillations recorded as a frequency spectrogram (left) and amplitude (right) during ball game and rest. c , Flexible wearable OPM-MEG helmet with 63 sensor mounts. d , SQUID-MEG (top) and OPM-MEG (bottom) recording of 11-year-old patient with refractory focal epilepsy. Left: superimposed data of multiple sensors showing filtered background brain activity and interictal epileptiform discharges (IEDs). Right: averaged IED data and magnetic field topography at the spike peak. e , OPM-magnetocardiography (MCG) measured at ambient conditions with a 87 Rb magnetic gradiometer. Parts a , b adapted with permission from ref. 4 , Springer Nature Ltd. Part c adapted with permission from ref. 149 under a Creative Commons licence CC BY 4.0 . Part d adapted with permission from ref. 53 , RSNA. Part e adapted with permission from ref. 51 , APS.
To detect the weak magnetic signal originating from the brain, it is crucial to suppress much stronger magnetic-field backgrounds. Such suppression is achieved through magnetic shielding and fast field compensation with electromagnetic coils 47 , 48 , 49 , 50 . This approach, combined with the light weight (about 1 kg) of an OPM-MEG helmet, has enabled head movement up to 10 cm during human MEG recording 4 . It also allowed the subject to bounce a ball off a bat or to rotate their head while a MEG signal was recorded 4 (Fig. 3b ). MEG detection has also been demonstrated at ambient conditions without magnetic shielding by combining two OPM magnetometers into a first-order gradiometer 51 .
Another key advantage of OPMs not needing cryogenics is that the MEG helmet can be made to fit any head size (Fig. 3c ). This possibility is especially helpful for neuroimaging of infants and enables long-term studies as the infants grow 52 . Subjects who are intimidated by the bulky SQUID-based MEG helmet, and others who cannot stay still during the imaging procedure, could also get access to MEG scans.
OPM-based MEGs are increasingly finding clinical application. OPMs have been applied to detect and localize focal interictal spikes in children with epilepsy 53 (Fig. 3d ). For many neuroscientific studies it is also important to detect signal from deeper within the brain. One example is the human hippocampus, which is crucial for navigation and has been studied with OPM-based MEGs 54 , 55 . Another proof-of-concept demonstration has been the detection of functional connectivity with a 50-channel OPM 56 , paving the way for studies of brain networks. Moreover, OPMs have successfully been used to measure human retinal activity 57 ; the use of OPMs is contactless, in contrast to the fibre electrodes currently used. Another exciting demonstration of OPM-MEG has been the detection of human visual gamma-band activity in the brain 58 . These high-frequency oscillations are assumed to play an important role in cognitive functions. The high spatial resolution of OPMs aids the localization of the active source areas, which is challenging with currently used techniques 58 . OPMs will also enable new possibilities in functional neuroimaging. For example, neuroscientists can now monitor brain activity while the subject moves and performs tasks of interest 4 . One can even envisage combining OPM-MEG with virtual-reality devices 59 .
OPM-MEG is a relatively new technique, and further improvements are required before it can achieve widespread use in hospitals. Currently, the noise floor measured with OPMs is higher than SQUIDs by a factor of three, negating the sensitivity increase coming from smaller sensor-to-sample distance. So far, <100 OPM sensors have been combined into an array for MEG measurements 60 ( https://www.cercamagnetics.com/cerca-opm-meg ), in contrast to typical SQUID-MEG arrays of ~300 sensors. Scaling up the OPM sensor array, to provide comparable or superior resolution to SQUID-based MEG, will require the suppression of crosstalk between individual OPMs. The OPM community is working on these challenges and moving towards commercialization.
Another notable application of OPMs is MCG 18 , 51 (Fig. 3e ). In this case, the measurement of magnetic fields from the heart allows diagnosis of diverse heart conditions associated with electrically active cardiac cells. The main advantages of OPM-MCG, relative to SQUID-based detectors, are its portability, low cost, and electrode-free application 61 . Some commercial OPM-MCG devices are already available ( https://genetesis.com ).
NV-based magnetic sensing and imaging of cells and tissues
Individual cells and tissues can produce magnetic fields, as exemplified by the fields produced by neuronal action potentials or by chains of magnetic nanoparticles (magnetosomes) in bacteria. Magnetic tags can also be introduced into living systems, in the form of magnetic nanoparticles (MNPs) or spin labels, for example. In all these cases, a biocompatible magnetometer with high sensitivity and high spatial resolution is required to measure the fields. One common method for such investigations uses a millimetre-scale diamond chip with a thin surface layer (micrometre scale) of ensemble NV centres 62 . Another commonly used modality is nanodiamonds containing NV centres, which can be injected or ingested into cells or tissues and functionalized, to target proteins of interest, for example.
Magnetic nanoparticle imaging
Labelling, detecting and targeting individual cells is useful for diagnostic applications, such as distinguishing cancer cells from healthy cells. Fluorescent markers are commonly used as labels, but often suffer from blinking, photobleaching and background autofluorescence. MNPs form the basis of magnetic immunoassay techniques, an emerging complementary diagnostic modality with potential advantages over fluorescent markers: long-term stability, negligible background signal and quantitative detection.
Diamond NV centres have been used for quantitative detection and wide-field imaging of MNPs in diverse biological samples, with micrometre-scale resolution and a millimetre field of view. In an early demonstration, NV-based magnetic microscopy was used to resolve and quantitatively characterize chains of MNPs (magnetosomes) that occur naturally in magnetotactic bacteria 62 . NV-based magnetic microscopy was also used to detect cancer biomarkers 63 (Fig. 4a , b ). In this study, SKBR3 cancer cells were labelled with HER2-specific MNPs (where HER2 is human epidermal growth factor receptor 2), allowing differentiation between healthy and cancer cells. The technique is now commercially available for diagnostic assessment of biomarkers in human blood and other samples ( https://qdti.com/ ). In another demonstration, NV-diamond magnetic microscopy was used to investigate malarial haemozoin nanocrystals, which serve as biomarkers for malaria 64 (Fig. 4c ). These nanocrystals are formed in human blood cells infected by Plasmodium falciparum . In this study, the paramagnetic nature of the haemozoin nanocrystals was confirmed, and a magnetic susceptibility of 3.4 × 10 –4 was measured, opening a possibility of drug screening against cells infected by Plasmodium .
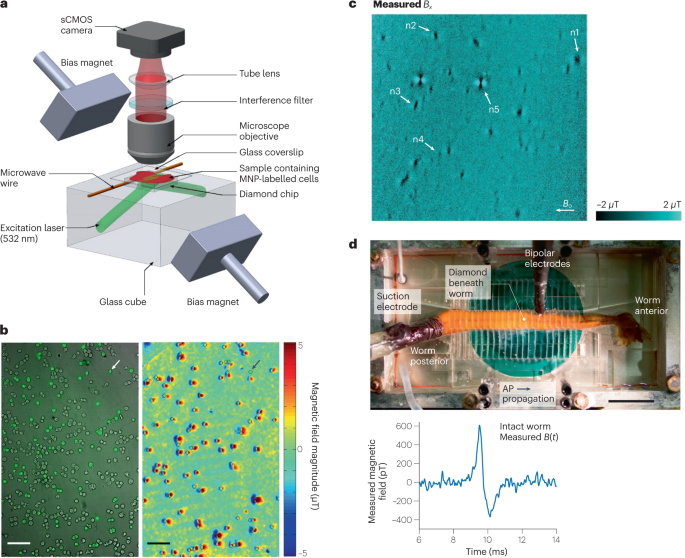
a , Wide-field NV-diamond microscope for magnetic imaging of cells. sCMOS, scientific complementary metal–oxide–semiconductor. b , Wide-field imaging of biomarkers. Left: bright-field image overlayed with fluorescence image of SKBR3 cancer cells labelled with magnetic nanoparticles (MNPs) and stained with fluorescence dyes. Right: same field of view showing NV magnetic imaging of MNP-labelled cells. Scale bar, 100 µm. c , Magnetic field image of natural haemozoin crystals acquired with a NV-diamond microscope. Field of view is 39 × 39 µm². d , Top: image of NV-diamond set-up for single-neuron action potential (AP) magnetic measurement of a living specimen of Myxicola infundibulum (worm). Bottom: time trace of the magnetic field signal coming from a single-neuron action potential of M. infundibulum detected with the NV-diamond set-up. Part a adapted with permission from ref. 62 , Springer Nature Ltd. Part b adapted with permission from ref. 63 , Springer Nature Ltd. Part c adapted with permission from ref. 64 under a Creative Commons licence CC BY 4.0 . Part d adapted with permission from ref. 66 , PNAS.
In another demonstration, NV-based magnetic microscopy was applied to detect concentrations of magnetically labelled protein, interleukin-6 65 , from patients hospitalized with COVID-19. Interleukin-6 is an endogenous cytokine associated with various diseases, such as severe COVID-19. The key idea was to detect the concentration of magnetic beads in the sample, and the results correlated well with the well-established Luminex assay technique. This success demonstrates the potential of NV-based microscopy for applications including rapid clinical testing.
Single-neuron action potential measurement
MEG is a powerful tool to investigate brain activity on macroscopic scales by interpreting effective current dipole sources in vivo. It would be desirable to understand how these effective current dipoles are related to underlying neuronal circuits. Such studies require minimally invasive magnetic measurements, ranging from single cells at the microscale to full neuronal circuits at the millimetre scale. NV-based microscopy has been used for a proof-of-concept measurement towards that direction. In this study, shallowly implanted diamond NV centres were used to measure the action potentials of single neurons from marine worms and squid 66 (Fig. 4d ). The study achieved a magnetic field sensitivity of ~10 pT Hz −1/2 . Importantly, the submillisecond time resolution of the technique allowed the direct measurement of the action potential waveforms, including the direction of action potential propagation along the neuron and detection in whole, live animals. These studies at both the single-neuron and neuronal-circuit levels would improve and validate assumptions used in MEG for current dipole source reconstruction and could potentially improve the resolution of MEG as a result.
Detection of spin labels with T1 relaxometry
The measurement of the longitudinal relaxation time (T1) of NVs is sensitive to magnetic noise at the NV spin-transition frequency, from megahertz to the gigahertz regime. This method can be applied to enable highly sensitive detection of magnetic ions such as Gd 3+ , the most common contrast agent used in magnetic resonance 67 . NV centres are allowing studies with these contrast agents down to the scale of individual cells, such as the detection and imaging of the plasma membrane of a HeLa cell labelled with Gd 3+ ions, with a spatial resolution of 400 nm (ref. 68 ). The resolution of NV T1 relaxometry can be further improved with a NV scanning probe set-up, as demonstrated by a spatial resolution of 10 nm for intracellular ferritin in Hep G2 cells 69 .
Biomagnetic sensing with nanodiamonds
Bringing very small biological samples (such as organelles within cells) close to the NV centres located in a macroscopic diamond chip can be challenging. Nanodiamonds containing NV centres are being investigated as an alternative approach for such applications. Nanodiamonds can be inserted into the interior of cells, tissue and other biological samples. Functionalization of the nanodiamond surface can also enable targeting to proteins or other biological targets of interest. As the NV axis is randomly oriented for nanodiamonds, it is difficult to perform sensitive NV magnetometry using coherent techniques such as optically detected magnetic resonance (ODMR). However, NV T1 relaxometry can still be used, thus allowing the detection of metalloproteins 70 , Gd 3+ spin-labelled lipid bilayers 71 and the rotational Brownian motion of spin-labelled molecules 72 .
A further application of T1 relaxometry with NV centres in nanodiamonds is the nanoscale detection of free radicals in biological samples. Formation of free radicals is linked to cardiovascular diseases and neurological disorder 73 . Free radicals can also play a vital role for the immune system 74 . Their sensitive detection with subcellular spatial resolution can therefore have far-reaching consequences in understanding biological processes. T1 relaxometry with NV centres in nanodiamonds makes such detection possible and has recently been used to study free radicals in single mitochondria 75 and in human dentritic cells 76 . In these experiments, the challenge is to carefully analyse the effect of free radicals on the T1 of the NV and rule out other influences. Another promising nanodiamond application is in vivo thermometry, which is discussed below.
Challenges and outlook
A key challenge for NV-based magnetic sensing and imaging of cells and tissues is to improve the sensitivity, which can lead to faster measurements and enable real-time mapping of neuronal activity and real-time functional imaging of biological samples, for example. Here we discuss some key opportunities for improved sensitivity 9 : improved readout techniques, which includes increasing the readout fidelity and minimizing the NV spin-state initialization and readout times; improved sample quality, which includes increasing the number of sensor spins per unit diamond sensing volume and extending the dephasing time; and improved measurement protocols, such as double quantum magnetometry 77 .
Techniques to improve readout fidelity include spin-to-charge conversion 78 , repetitive readout using nuclear spins coupled to the NV as quantum memories 79 , and enhanced photon collection through various forms of diamond fabrication, tailored lenses or light guides 80 . Although these techniques have been highly successful for single NV measurements, more work is required for their adaptation to large-scale diamond chips with NV ensembles.
On the material side, high-density NV ensembles have typically been grown with high-pressure, high-temperature methods. More recently, high-quality chemical vapour deposition (CVD) samples have been prepared, including growth of thin nitrogen layers on relatively nitrogen-free substrates. To maximize NV creation in such layers, vacancy creation via electron irradiation is typically performed, resulting in NV ensembles with densities up to 4 ppm (ref. 81 ). Unfortunately, the CVD growth process for high NV densities typically introduces additional paramagnetic defects and strain, affecting the coherence time of the sensor. Methods to mitigate these effects are being developed 82 , 83 . For NV centres within a few tens of nanometres of the diamond surface, surface-related charge instabilities and noise further degrade NV properties. Approaches to address this problem are actively being explored, including careful surface cleaning and termination 84 , and delta doping of NVs, in which NVs are created in a thin surface layer and an additional diamond layer is then overgrown 85 . Another challenge in biomedical applications is reproducibility of results. To meet this challenge, it will be important to develop recipes to clean the diamond surface and maintain the coherence properties of the shallow NV centres in multiple rounds of experiments.

Nano- and microscale NMR with NV centres
NMR is a spectroscopic tool widely used in chemistry, biology and medicine for the structural determination of organic and biological molecules 86 . The technique usually relies on the detection of thermally polarized nuclear spins; to do so, large magnetic fields of the order of several tesla need to be applied 87 , 88 . A major limitation of traditional NMR is its low sensitivity, typically requiring millimetre-scale samples.
Extending NMR spectroscopy to microscale and nanoscale samples would enable exciting applications. It would, for example, allow chemical analysis of mass-limited samples that are expensive or difficult to synthesize. Furthermore, NMR spectroscopy of a single-cell volume could enable detailed studies of cell structure and function, with applications in metabolomics and disease diagnosis 89 , 90 . Bringing NMR down to the nanometre scale would also make single-protein detection possible, opening up the possibility of determining the structure of functional membrane proteins under near-physiological conditions and studying their dynamics 91 . Membrane proteins are the targets of more than half of FDA-approved drugs 92 , and the real-time study of protein–molecule binding would aid drug discovery.
NV-based NMR
The advent of NV-based magnetometry has enabled NMR spectroscopy of nanoscale and microscale samples under ambient conditions 6 , 93 (Fig. 5a ). Here, the sample is placed near the NV centres in diamond, with distances in the range of nanometres to micrometres depending on the specific application 6 , 94 (Fig. 5a ). At the nanoscale, NV-based NMR benefits from the large statistical polarization of the sample spins, while on the microscale, thermal polarization dominates 95 , often necessitating further enhancements via high magnetic fields 96 and hyperpolarization methods 97 , 98 . One feature of NV centres is their large magnetic field sensing bandwidth, ranging from d.c. to gigahertz 7 . Detection of multiple nuclear spin species 99 , 100 and even electron spins 101 is therefore possible with the same experimental set-up, without the need of changing the radiofrequency equipment as is the case for traditional NMR and electron paramagnetic resonance spectroscopy.
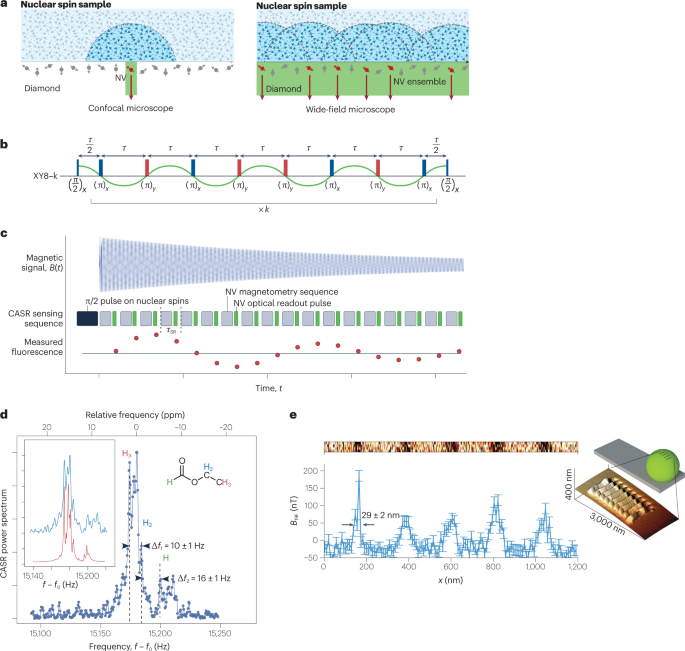
a , Single-NV and NV-ensemble NMR set-ups. b , c , Pulse sequences used for NMR detection with NV centres: XY8-k (XY8 is a sequence of microwave pulses with X and Y phase shifts and is repeated k times, part b ) and coherently averaged synchronized readout (CASR, part c ). d , NMR spectrum detected by NV ensembles showing resolved chemical shift. e , Inset: image of a grating etched on a fluorine-enriched microsphere on an atomic force microscope tip. Main image: line scan of the NMR spectrum detected by a single NV centre as the sphere is moved over the NV centre. Parts a , b adapted with permission from ref. 100 , Springer Nature Ltd. Parts c , d adapted with permission from ref. 6 , Springer Nature Ltd. Part e adapted with permission from ref. 115 , Springer Nature Ltd.
Figures of merit
For NV-based NMR, the key figures of merit are the spatial resolution, spectral resolution and sensitivity of the spectrometer. The spatial resolution, or sensing volume, is of the order of the (depth) 3 of the NV centres, typically ranging from (4 nm)³ to (10 µm)³, and can be chosen based on the specific application. For NV-ensemble measurements, the spatial resolution is further limited by the laser spot size. The sensitivity, conversely, follows similar considerations to those discussed in the previous section on NV-based magnetic sensing. At the nanoscale, single-proton spin detection in 1-s integration has been demonstrated 94 ; whereas at the microscale, the detection of ~10 14 thermally polarized proton spins (~10 picolitre) has been demonstrated with 1-s integration 6 . The sensitivity can be further improved via hyperpolarization, as discussed below.
To resolve molecule-specific resonances, such as chemical shifts and J -couplings, a high spectral resolution of around one part per million (of the order of hertz in absolute frequency units) is required 102 . This resolution has been realized using different techniques 5 , 6 . The achievable spectral resolution is limited by both the NV centre and the sample. The limitation from the NV centre depends on the applied sensing sequence: dynamical decoupling (Fig. 5b ) can achieve a spectral resolution of ~1 kHz, limited by the NV T2 time 93 . Correlation spectroscopy can further improve the resolution to ~100 Hz, limited by the NV T1 time 99 . Even further enhanced spectral resolution can be reached with memory-spin-enhanced sensing 5 , 103 , limited by the T1 of the NV nitrogen nuclear spin, which can be >260 s (ref. 103 ). Another approach is to coherently average a sensing step that is then synchronized to an external clock 6 , 104 , 105 (Fig. 5c ).
On the sample side, physical diffusion (liquids) or spin diffusion (solids) is often the limiting factor. Fast translational diffusion in liquids limits the spectral resolution to ~1 kHz for viscous liquids and makes liquids with low viscosity undetectable 100 . This problem can be addressed by confining samples into a nanoscale volume 106 , 107 . For solid-state samples, dipole–dipole broadening is the limiting factor 94 , and heteronuclear and homonuclear decoupling can help to improve the resolution 5 . Additionally, NV-based multidimensional NMR can help in reconstructing the chemical structure of a biomolecule. In a proof-of-principle experiment, 27 13 C spins were localized in a diamond lattice relative to a single NV centre 108 . NV-based NMR has also been applied to samples external to the diamond, as demonstrated by the detection of a single protein with a spectral resolution of 1 kHz (ref. 94 ).
Hyperpolarization
Spin polarization beyond thermal equilibrium, typically achieved by transferring polarization from more readily polarizable electronic spins to the target nuclear spins, can enhance the NMR signal and thus improve detection sensitivity. Dynamic nuclear polarization, based on the Overhauser mechanism 97 , and parahydrogen-based signal amplification by reversible exchange (SABRE) 98 were used to hyperpolarize proton spins to 0.5%, leading to a signal enhancement of NV-based NMR of about 2 × 10 5 and the detection of target molecules at concentrations as low as 1 millimolar 98 . The NV spins can also serve as a source of hyperpolarization, because they can be well polarized via optical pumping (Fig. 2b ). Through this technique, surrounding 13 C nuclear spins were polarized to ~720 times the thermal value at 7 T (refs. 109 , 110 , 111 ). Unfortunately, no external spin hyperpolarization has yet been demonstrated. This is due to low diamond surface-to-volume ratios 111 , decreased spin coherence times of near-surface NVs 112 , 113 and short nuclear spin T1 times close to the diamond surface 84 , 114 . Laboratories worldwide are working to address these challenges, by better control of the diamond surface and by achieving high densities of shallow NVs with improved coherence properties, for example 81 , 112 , 113 .
Nanoscale and microscale magnetic resonance imaging
The full potential of NV-based NMR unfolds when imaging biological samples, as it can reveal changes in chemical composition at the nanoscale to microscale (Fig. 5d ). At the microscale, magnetic resonance imaging (MRI) can be realized by a wide-field set-up with an ensemble of NV centres 100 , and scanning probe systems can be used for MRI with higher spatial resolution of the order of ~10 nm (ref. 115 ) (Fig. 5e ).
Owing to the high sensitivity on the nanoscale of NV centres, NVs are also considered a promising tool for studying surface chemistry. Functionalization of the diamond surface is of great importance for these applications, because it immobilizes molecules close to NV centres and enables surface NMR detection with NV centres 116 , 117 , 118 .
Future developments
It is well established that NV-based sensors can perform NMR spectroscopy of nanoscale and microscale samples and, under certain conditions, detect chemical shifts and J -couplings. Despite this progress, several major challenges remain for NV-based NMR. Incorporation into microfluidics is necessary for high-throughput NMR screening of certain samples, such as those that are mass-limited; higher sensitivities are required for structure determination of individual molecules; deterministic single (bio)molecule placement near a single NV centre would be desirable, possibly via scanning probe techniques 115 or surface treatment 116 , 117 , 118 ; and finally, increased spectral resolution by reducing sample diffusion 106 , 107 and designing new pulse sequences 83 will be highly beneficial.
With these further developments, NV-based NMR will expand capabilities beyond conventional techniques and open many avenues in chemistry as well as molecular and cell biology. Nanoscale NV-based NMR, aided by multidimensional spectroscopy and spin-labelling techniques, will enable the structural determination of complex molecules, such as transmembrane proteins in near-physiological conditions. Furthermore, wide-field NV-based MRI with its subcellular spatial resolution could be applied for single-cell metabolomics studies. Another application is to correlate NV-based NMR with optical microscopy of fluorophore labels, which can be useful for single-molecule studies. Ultimately, the goal would be to make these techniques accessible to non-physicists. Towards this goal, miniaturization of the tool is important. With recent developments 119 , 120 and further integration, chip-scale NV spectrometers may become a reality soon.
NV thermometry
Nv-based quantum thermometry.
Whereas the preceding sections focused on applications to magnetic field sensing, quantum sensors can also be sensitive to a range of other environmental influences 10 , 27 , 28 , 31 , 32 , 121 , 122 , 123 , 124 , 125 , providing rich sensing modalities relevant to the life sciences. In this section, we discuss one biological application of quantum sensors beyond magnetic field sensing: in vivo nanoscale thermometry with NVs in nanodiamonds. This modality enables local probing of a wide range of temperature-related biological phenomena in cells and small organisms, including the effects of external heat gradients and internal heat generation, providing tools for the control of cell cycles and organism development.
Like magnetic field sensing, NV-based quantum thermometry relies on temperature-dependent changes in microwave transition frequencies (Fig. 6a ) that originate from thermal expansion of diamond. The vibronic interactions between the NV spin and the host lattice result in a temperature-dependent zero-field splitting 124 , with a slope of approximately –74 kHz K −1 close to room temperature 31 , 121 , 122 , 123 , 124 . To optimize sensitivity while minimizing susceptibility to other effects, a four-point measurement scheme is typically used (Fig. 6b ). The temperature can also be measured via purely optical means through changes in the emission spectrum and intensity 28 , 125 , thus providing multimodal verification of results.
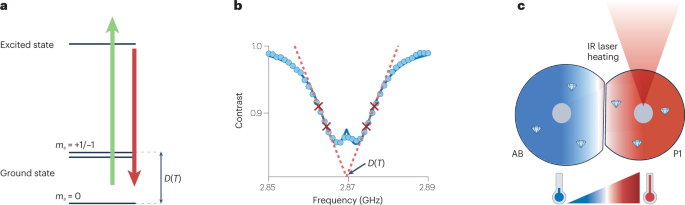
a , NV energy-level diagram with spin quantum number m s = 0,±1 and temperature-dependent zero-field splitting, D ( T ), which is typically used for thermometry. b , Four-point measurement scheme for noise-robust determination of temperature using NVs. c , Heat gradients between two cells AB and P1 at the early embryo stage, generated by localized heating using an infrared (IR) laser. Parts b,c adapted with permission from ref. 137 , PNAS.
Nanodiamond quantum sensors are well suited for high-spatial-resolution temperature sensing in cells and small organisms. Compared with conventional temperature probes, nanodiamond quantum sensors are nanoscale, stable and biocompatible 126 , 127 , 128 , 129 . They also provide a complementary tool to luminescent nanoscale thermometry performed with fluorescent nanoparticles or proteins 130 , which suffer from calibration issues, bleaching or susceptibility to frequency-dependent optical transmission 131 . Quantum sensing instead uses coherence between microwave transitions, reducing the sensitivity to optical transmission and other environmental factors.
NV centres can be created in nanodiamonds 10 , 31 , 70 , 71 , 72 , 127 , 128 , 129 , often in the form of NV ensembles to further increase sensitivity. The size of such nanodiamonds is typically around 50–100 nm, although smaller ones are available with inferior optical and spin properties 132 . With suitable surface treatment, these nanodiamonds can be delivered into individual cells. For some cell types, such as HeLa cells, natural uptake is sufficient, whereas for others, such as egg cells, injection techniques may be preferred 128 , 129 . Although suitably treated nanodiamonds have mostly been found to have no or low cytotoxicity, some studies suggest they can remain in organs for long periods 133 , motivating further long-term studies of their effects 128 , 134 . The NV temperature sensitivity is ~5 mK Hz −1/2 in isotopically purified, ultrapure bulk diamond 123 . The sensitivity in the nanodiamond form is poorer, however, because of strain effects and surface contamination. In a typical biological setting, the temperature sensitivity of NV nanodiamonds is ~1 K Hz −1/2 (refs. 31 , 135 , 136 , 137 ), comparable to other techniques 130 .
Applications of nanoscale thermometry
Sensitive nanoscale thermometry opens many possibilities in life science applications, especially in combination with localized exogenous heating induced by infrared laser illumination (Fig. 6c ). Laser heating has been used to explore the direct biochemical effects of elevated temperature (such as accelerated cell growth or protein denaturation) 31 , 135 , 138 , 139 , the expression of heat-shock protein promoters 140 , 141 , 142 , and thermal effects on cell and organism development. In an early proof-of-principle experiment that combines infrared heating and nanodiamond sensors, the threshold heating temperature that induces HeLa cell death was investigated 31 . More recently, local temperature manipulation and monitoring was used to control and invert the cell development cycle during embryogenesis in Caenorhabditis elegans 137 . Nanodiamonds have also been explored as a tool for in vivo temperature calibration for thermotherapeutic treatments 138 , 140 . Other possible applications of NV-based thermometry include nanoscale measurements of heat conductivity 143 , and endogenous heat generation 135 , 144 , 145 , 146 .
Although current techniques have yielded impressive demonstrations and shed light on the role of temperature on biological processes, considerable challenges remain. First, to measure absolute temperature changes accurately, it is necessary to perform precise in vivo calibration of quantum sensors in the presence of internal strain, stray magnetic fields, spatial movement in cells, and microwave and optical heating. Second, although techniques for incorporating nanodiamonds into living cells are fairly mature, more work is required on surface functionalization and nanodiamond synthesis to target specific organelles at the subcellular level 147 . Third, despite the fact that the temperature sensitivity of nanodiamond sensors is competitive with other technologies, further improvements are desired. These improvements include using hybrid nanodiamond–MNP schemes 148 , material improvements, spin echo techniques for T2-limited NV coherence measurements 31 , 131 , 132 (instead of the current T2*-limited measurements) and advanced readout methods 9 .
The field of quantum sensors has developed tremendously in the past decade, moving from early proof-of-principle experiments into real-world applications in biomedical sciences. Indeed, these developments have already spurred the creation of several start-up companies making use of the technologies discussed in the case studies above. Examples (not exhaustive) include: QuSpin, CercaMagnetics and FieldLine Inc., focusing on commercializing OPM-MEG technology; ODMR Technologies, focusing on NV-based magnetic resonance spectroscopy for chemical trace analysis; Quantum Diamond Technologies Inc. (QDTI), focusing on wide-field NV magnetic imaging of disease biomarkers; and NVision Imaging Technologies, focusing on NV-based hyperpolarization of nuclear spins for molecular analysis and medical imaging.
Although there are promising opportunities, many challenges remain, probably calling for collaborations between multiple academic domains and industry. On the one hand, as discussed in the case studies for NV-based optical magnetic imaging and NMR spectroscopy, the sensitivities of current quantum sensors will probably need to be further improved through a combination of new sensing protocols and material developments. On the other hand, further integration and miniaturization of such technologies, to enable scalability and ease of operation under realistic conditions, will be crucial for broad acceptance and commercial success. With these improvements, we expect quantum sensors to become key tools for characterization and diagnostics of biomedical systems.
Arute, F. et al. Quantum supremacy using a programmable superconducting processor. Nature 574 , 505–510 (2019).
Article ADS Google Scholar
Zhong, H.-S. et al. Quantum computational advantage using photons. Science 370 , 1460–1463 (2020).
Bhaskar, M. K. et al. Experimental demonstration of memory-enhanced quantum communication. Nature 580 , 60–64 (2020).
Boto, E. et al. Moving magnetoencephalography towards real-world applications with a wearable system. Nature 555 , 657–661 (2018).
Aslam, N. et al. Nanoscale nuclear magnetic resonance with chemical resolution. Science 357 , 67–71 (2017).
Glenn, D. R. et al. High-resolution magnetic resonance spectroscopy using a solid-state spin sensor. Nature 555 , 351–354 (2018).
Degen, C. L., Reinhard, F. & Cappellaro, P. Quantum sensing. Rev. Mod. Phys. 89 , 035002 (2017).
Article MathSciNet ADS Google Scholar
Budker, D. & Romalis, M. Optical magnetometry. Nat. Phys. 3 , 227–234 (2007).
Article Google Scholar
Barry, J. F. et al. Sensitivity optimization for NV-diamond magnetometry. Rev. Mod. Phys. 92 , 015004 (2020).
Schirhagl, R. et al. Nitrogen-vacancy centers in diamond: nanoscale sensors for physics and biology. Annu. Rev. Phys. Chem. 65 , 83–105 (2014).
Simmonds, M., Fertig, W. & Giffard, R. Performance of a resonant input SQUID amplifier system. IEEE Trans. Magn. 15 , 478–481 (1979).
Balabas, M. V. Polarized alkali-metal vapor with minute-long transverse spin-relaxation time. Phys. Rev. Lett. https://doi.org/10.1103/PhysRevLett.105.070801 (2010).
Dang, H. B., Maloof, A. C. & Romalis, M. V. Ultrahigh sensitivity magnetic field and magnetization measurements with an atomic magnetometer. Appl. Phys. Lett. 97 , 151110 (2010).
Shah, V. et al. Subpicotesla atomic magnetometry with a microfabricated vapour cell. Nat. Photon. 1 , 649–652 (2007).
Fernholz, T. et al. Spin squeezing of atomic ensembles via nuclear-electronic spin entanglement. Phys. Rev. Lett. 101 , 073601 (2008).
Wasilewski, W. et al. Quantum noise limited and entanglement-assisted magnetometry. Phys. Rev. Lett. 104 , 133601 (2010).
Xu, S. et al. Magnetic resonance imaging with an optical atomic magnetometer. Proc. Natl Acad. Sci. USA 103 , 12668–12671 (2006).
Jensen, K. et al. Magnetocardiography on an isolated animal heart with a room-temperature optically pumped magnetometer. Sci. Rep. 8 , 16218 (2018).
Deans, C. et al. Electromagnetic induction imaging with a radio-frequency atomic magnetometer. Appl. Phys. Lett. 108 , 103503 (2016).
Jensen, K. et al. Detection of low-conductivity objects using eddy current measurements with an optical magnetometer. Phys. Rev. Res. 1 , 033087 (2019).
Balasubramanian, G. et al. Nanoscale imaging magnetometry with diamond spins under ambient conditions. Nature 455 , 648–651 (2008).
Maze, J. R. et al. Nanoscale magnetic sensing with an individual electronic spin in diamond. Nature 455 , 644–647 (2008).
Gruber, A. et al. Scanning confocal optical microscopy and magnetic resonance on single defect centers. Science 276 , 2012–2014 (1997).
Balasubramanian, G. et al. Ultralong spin coherence time in isotopically engineered diamond. Nat. Mater. 8 , 383–387 (2009).
Toyli, D. M. et al. Measurement and control of single nitrogen-vacancy center spins above 600 K. Phys. Rev. X 2 , 031001 (2012).
Google Scholar
Fortman, B. et al. Electron–electron double resonance detected NMR spectroscopy using ensemble NV centers at 230 GHz and 8.3 T. J. Appl. Phys. 130 , 083901 (2021).
Hsieh, S. et al. Imaging stress and magnetism at high pressures using a nanoscale quantum sensor. Science 366 , 1349–1354 (2019).
Nguyen, C. T. et al. All-optical nanoscale thermometry with silicon-vacancy centers in diamond. Appl. Phys. Lett. 112 , 203102 (2018).
Bradley, C. E. et al. A ten-qubit solid-state spin register with quantum memory up to one minute. Phys. Rev. X 9 , 031045 (2019).
Zhang, C. et al. Diamond magnetometry and gradiometry towards subpicotesla DC field measurement. Phys. Rev. Appl. 15 , 064075 (2021).
Kucsko, G. et al. Nanometre-scale thermometry in a living cell. Nature 500 , 54–58 (2013).
Dolde, F. et al. Electric-field sensing using single diamond spins. Nat. Phys. 7 , 459–463 (2011).
Schäfer-Nolte, E. et al. Tracking temperature-dependent relaxation times of ferritin nanomagnets with a wideband quantum spectrometer. Phys. Rev. Lett. 113 , 217204 (2014).
Maletinsky, P. et al. A robust scanning diamond sensor for nanoscale imaging with single nitrogen-vacancy centres. Nat. Nanotechnol. 7 , 320–324 (2012).
Koehl, W. F. et al. Room temperature coherent control of defect spin qubits in silicon carbide. Nature 479 , 84–87 (2011).
Kornher, T. et al. Sensing individual nuclear spins with a single rare-earth electron spin. Phys. Rev. Lett. 124 , 170402 (2020).
Williamson, S. J. & Manfried H. Advances in Biomagnetism (eds Williamson, S. J. et al.) (Springer, 2012).
Allen, C. M. et al. Magnetoencephalography abnormalities in adult mild traumatic brain injury: a systematic review. NeuroImage Clin. 31 , 102697 (2021).
Poghosyan, V., Rampp, S. & Wang, Z. I. Editorial: magnetoencephalography (MEG) in epilepsy and neurosurgery. Front. Human Neurosci . https://doi.org/10.3389/fnhum.2022.873153 (2022).
Hughes, L. E. et al. Magnetoencephalography insights to dementia and drug intervention. Alzheimer’s Dement. 17 , e052536 (2021).
Cohen, D. Magnetoencephalography: detection of the brain’s electrical activity with a superconducting magnetometer. Science 175 , 664–666 (1972).
Hämäläinen, M. et al. Magnetoencephalography — theory, instrumentation, and applications to noninvasive studies of the working human brain. Rev. Mod. Phys. 65 , 413–497 (1993).
QZFM Gen-3. QUSPIN https://quspin.com/products-qzfm/ .
Rea, M. et al. A 90-channel triaxial magnetoencephalography system using optically pumped magnetometers. Ann. NY Acad. Sci. 1517 , 107–124 (2022).
Brookes, M. J. et al. Theoretical advantages of a triaxial optically pumped magnetometer magnetoencephalography system. NeuroImage 236 , 118025 (2021).
Boto, E. et al. Triaxial detection of the neuromagnetic field using optically-pumped magnetometry: feasibility and application in children. NeuroImage 252 , 119027 (2022).
Rea, M. et al. Precision magnetic field modelling and control for wearable magnetoencephalography. NeuroImage 241 , 118401 (2021).
Iivanainen, J. et al. On-scalp MEG system utilizing an actively shielded array of optically-pumped magnetometers. NeuroImage 194 , 244–258 (2019).
Mäkinen, A. J. et al. Magnetic-field modeling with surface currents. Part I. Physical and computational principles of bfieldtools. J. Appl. Phys. 128 , 063906 (2020).
Holmes, N. et al. A bi-planar coil system for nulling background magnetic fields in scalp mounted magnetoencephalography. NeuroImage 181 , 760–774 (2018).
Limes, M. E. et al. Portable magnetometry for detection of biomagnetism in ambient environments. Phys. Rev. Appl. 14 , 011002 (2020).
Hill, R. M. et al. A tool for functional brain imaging with lifespan compliance. Nat. Commun. 10 , 4785 (2019).
Feys, O. et al. On-scalp optically pumped magnetometers versus cryogenic magnetoencephalography for diagnostic evaluation of epilepsy in school-aged children. Radiology 304 , 429–434 (2022).
Barry, D. N. et al.Imaging the human hippocampus with optically-pumped magnetoencephalography. NeuroImage 203 , 116192 (2019).
Tierney, T. M. et al. Mouth magnetoencephalography: a unique perspective on the human hippocampus. NeuroImage 225 , 117443 (2021).
Boto, E. et al. Measuring functional connectivity with wearable MEG. NeuroImage 230 , 117815 (2021).
Westner, B. U. et al. Contactless measurements of retinal activity using optically pumped magnetometers. NeuroImage 243 , 118528 (2021).
Iivanainen, J., Zetter, R. & Parkkonen, L. Potential of on-scalp MEG: robust detection of human visual gamma-band responses. Hum. Brain Mapp. 41 , 150–161 (2020).
Roberts, G. et al. Towards OPM-MEG in a virtual reality environment. NeuroImage 199 , 408–417 (2019).
Hill, R. M. et al. Multi-channel whole-head OPM-MEG: Helmet design and a comparison with a conventional system. NeuroImage 219 , 116995 (2020).
Batie, M. et al. Detection of fetal arrhythmia using optically-pumped magnetometers. JACC Clin. Electrophysiol. 4 , 284–287 (2018).
Le Sage, D. et al. Optical magnetic imaging of living cells. Nature 496 , 486–489 (2013).
Glenn, D. R. et al. Single-cell magnetic imaging using a quantum diamond microscope. Nat. Methods 12 , 736–738 (2015).
Fescenko, I. et al. Diamond magnetic microscopy of malarial hemozoin nanocrystals. Phys. Rev. Appl. 11 , 034029 (2019).
Atallah, J. et al. Rapid quantum magnetic IL-6 point-of-care assay in patients hospitalized with COVID-19. Diagnostics 12 , 1164 (2022).
Barry, J. F. et al. Optical magnetic detection of single-neuron action potentials using quantum defects in diamond. Proc. Natl Acad. Sci. USA 113 , 14133–14138 (2016).
Lauffer, R. B. Paramagnetic metal complexes as water proton relaxation agents for NMR imaging: theory and design. Chem. Rev. 87 , 901–927 (1987).
Steinert, S. et al. Magnetic spin imaging under ambient conditions with sub-cellular resolution. Nat. Commun. 4 , 1607 (2013).
Wang, P. et al. Nanoscale magnetic imaging of ferritins in a single cell. Sci. Adv. 5 , eaau8038 (2019).
Ermakova, A. et al. Detection of a few metallo-protein molecules using color centers in nanodiamonds. Nano Lett. 13 , 3305–3309 (2013).
Kaufmann, S. et al. Detection of atomic spin labels in a lipid bilayer using a single-spin nanodiamond probe. Proc. Natl Acad. Sci. USA 110 , 10894–10898 (2013).
Li, C. et al. All-optical quantum sensing of rotational Brownian motion of magnetic molecules. Nano Lett. 19 , 7342–7348 (2019).
Lobo, V. et al. Free radicals, antioxidants and functional foods: impact on human health. Pharmacogn. Rev. 4 , 118–126 (2010).
Knight, J. A. Review: free radicals, antioxidants, and the immune system. Ann. Clin. Lab. Sci. 30 , 145–158 (2000).
Nie, L. et al. Quantum monitoring of cellular metabolic activities in single mitochondria. Sci. Adv. 7 , eabf0573 (2021).
Nie, L. et al. Quantum sensing of free radicals in primary human dendritic cells. Nano Lett. 22 , 1818–1825 (2022).
Mamin, H. J. et al. Multipulse double-quantum magnetometry with near-surface nitrogen-vacancy centers. Phys. Rev. Lett. 113 , 030803 (2014).
Shields, B. J. et al. Efficient readout of a single spin state in diamond via spin-to-charge conversion. Phys. Rev. Lett. 114 , 136402 (2015).
Arunkumar, N. et al. Quantum logic enhanced sensing in solid-state spin ensembles. Preprint at https://arxiv.org/abs/2203.12501 (2022).
Babinec, T. M. et al. A diamond nanowire single-photon source. Nat. Nanotechnol. 5 , 195–199 (2010).
Edmonds, A. M. et al. Generation of nitrogen-vacancy ensembles in diamond for quantum sensors: optimization and scalability of CVD processes. Preprint at https://arxiv.org/abs/2004.01746 (2020).
Joos, M. et al. Protecting qubit coherence by spectrally engineered driving of the spin environment. Preprint at https://arxiv.org/abs/2101.09654 (2021).
Zhou, H. et al. Quantum metrology with strongly interacting spin systems. Phys. Rev. X 10 , 031003 (2020).
Sangtawesin, S. et al. Origins of diamond surface noise probed by correlating single-spin measurements with surface spectroscopy. Phys. Rev. X 9 , 031052 (2019).
Ohno, K. et al. Engineering shallow spins in diamond with nitrogen delta-doping. Appl. Phys. Lett. 101 , 082413 (2012).
Mansfield, P. Snapshot magnetic resonance imaging (Nobel Lecture). Angew. Chem. Int. Ed. 43 , 5456–5464 (2004).
Glover, P. & Mansfield, S. P. Limits to magnetic resonance microscopy. Rep. Prog. Phys. 65 , 1489 (2002).
Abraham, R., Fisher, J. & Loftus, P. Introduction to NMR Spectroscopy (Wiley, 2022); https://www.wiley.com/en-gb/Introduction+to+NMR+Spectroscopy-p-9780471918943 .
Weisman, I. D. et al. Recognition of cancer in vivo by nuclear magnetic resonance. Science 178 , 1288–1290 (1972).
Lauterbur, P. C. Image formation by induced local interactions: examples employing nuclear magnetic resonance. Nature 242 , 190–191 (1973).
Fox, R. O., Evans, P. A. & Dobson, C. M. Multiple conformations of a protein demonstrated by magnetization transfer NMR spectroscopy. Nature 320 , 192–194 (1986).
Moraes, I. et al. Membrane protein structure determination — the next generation. Biochim. Biophys. Acta 1838 , 78–87 (2014).
Staudacher, T. et al. Nuclear magnetic resonance spectroscopy on a (5-nanometer) 3 sample volume. Science 339 , 561–563 (2013).
Lovchinsky, I. et al. Nuclear magnetic resonance detection and spectroscopy of single proteins using quantum logic. Science 351 , 836–841 (2016).
Article MathSciNet MATH ADS Google Scholar
Herzog, B. E. et al. Boundary between the thermal and statistical polarization regimes in a nuclear spin ensemble. Appl. Phys. Lett. 105 , 043112 (2014).
Smits, J. et al. Two-dimensional nuclear magnetic resonance spectroscopy with a microfluidic diamond quantum sensor. Sci. Adv. 5 , eaaw7895 (2019).
Bucher, D. B. et al. Hyperpolarization-enhanced NMR spectroscopy with femtomole sensitivity using quantum defects in diamond. Phys. Rev. X 10 , 021053 (2020).
Arunkumar, N. et al. Micron-scale NV-NMR spectroscopy with signal amplification by reversible exchange. PRX Quantum 2 , 010305 (2021).
Lovchinsky, I. et al. Magnetic resonance spectroscopy of an atomically thin material using a single-spin qubit. Science 355 , 503–507 (2017).
DeVience, S. J. et al. Nanoscale NMR spectroscopy and imaging of multiple nuclear species. Nat. Nanotechnol. 10 , 129–134 (2015).
Schlipf, L. et al. A molecular quantum spin network controlled by a single qubit. Sci. Adv. 3 , e1701116 (2017).
Schwartz, I. et al. Blueprint for nanoscale NMR. Sci. Rep. 9 , 6938 (2019).
Pfender, M. et al. Nonvolatile nuclear spin memory enables sensor-unlimited nanoscale spectroscopy of small spin clusters. Nat. Commun. 8 , 834 (2017).
Boss, J. M. et al. Quantum sensing with arbitrary frequency resolution. Science 356 , 837–840 (2017).
Schmitt, S. et al. Submillihertz magnetic spectroscopy performed with a nanoscale quantum sensor. Science 356 , 832–837 (2017).
Cohen, D. et al. Confined Nano-NMR spectroscopy using NV centers. Adv. Quantum Technol. 3 , 2000019 (2020).
Aslam, N. et al. Nanoscale nuclear magnetic resonance with quantum sensors enhanced by nanostructures. In Quantum 2.0 Conference and Exhibition (2022) Paper QW4C.3 (Optica Publishing Group, 2022).
Abobeih, M. H. et al. Atomic-scale imaging of a 27-nuclear-spin cluster using a quantum sensor. Nature 576 , 411–415 (2019).
Ajoy, A. et al. Room temperature ‘optical nanodiamond hyperpolarizer’: physics, design, and operation. Rev. Sci. Instrum. 91 , 023106 (2020).
Ajoy, A. et al. Orientation-independent room temperature optical 13 C hyperpolarization in powdered diamond. Sci. Adv. 4 , eaar5492 (2018).
Schwartz, I. et al. Robust optical polarization of nuclear spin baths using Hamiltonian engineering of nitrogen-vacancy center quantum dynamics. Sci. Adv. 4 , eaat8978 (2018).
Tetienne, J.-P. et al. Spin properties of dense near-surface ensembles of nitrogen-vacancy centers in diamond. Phys. Rev. B 97 , 085402 (2018).
Rubinas, O. R. et al. Optimization of the coherence properties of diamond samples with an intermediate concentration of NV centers. Results Phys. 21 , 103845 (2021).
Dhomkar, S. et al. Charge dynamics in near-surface, variable-density ensembles of nitrogen-vacancy centers in diamond. Nano Lett. 18 , 4046–4052 (2018).
Häberle, T. et al. Nanoscale nuclear magnetic imaging with chemical contrast. Nat. Nanotechnol. 10 , 125–128 (2015).
Liu, K. S. et al. Surface NMR using quantum sensors in diamond. Proc. Natl Acad. Sci. USA 119 , e2111607119 (2022).
Xie, M. et al. Biocompatible surface functionalization architecture for a diamond quantum sensor. Proc. Natl Acad. Sci. USA 119 , e2114186119 (2022).
Abendroth, J. M. et al. Single-nitrogen–vacancy NMR of amine-functionalized diamond surfaces. Nano Lett. 22 , 7294–7303 (2022).
Stürner, F. M. et al. Integrated and portable magnetometer based on nitrogen-vacancy ensembles in diamond. Adv. Quantum Technol. 4 , 2000111 (2021).
Ibrahim, M. I. et al. High-scalability CMOS quantum magnetometer with spin-state excitation and detection of diamond color centers. IEEE J. Solid State Circuits 56 , 1001–1014 (2021).
Sotoma, S., Epperla, C. P. & Chang, H.-C. Diamond nanothermometry. ChemNanoMat 4 , 15–27 (2018).
Toyli, D. M. et al. Fluorescence thermometry enhanced by the quantum coherence of single spins in diamond. Proc. Natl Acad. Sci. USA 110 , 8417–8421 (2013).
Neumann, P. et al. High-precision nanoscale temperature sensing using single defects in diamond. Nano Lett. 13 , 2738–2742 (2013).
Acosta, V. M. et al. Temperature dependence of the nitrogen-vacancy magnetic resonance in diamond. Phys. Rev. Lett. 104 , 070801 (2010).
Plakhotnik, T. et al. All-optical thermometry and thermal properties of the optically detected spin resonances of the NV – center in nanodiamond. Nano Lett. 14 , 4989–4996 (2014).
Mochalin, V. N. et al. The properties and applications of nanodiamonds. Nat. Nanotechnol. 7 , 11–23 (2012).
McGuinness, L. P. et al. Quantum measurement and orientation tracking of fluorescent nanodiamonds inside living cells. Nat. Nanotechnol. 6 , 358–363 (2011).
van der Laan, K. J. et al. Nanodiamonds for in vivo applications. Small 14 , 1703838 (2018).
Mohan, N. et al. In vivo imaging and toxicity assessments of fluorescent nanodiamonds in Caenorhabditis elegans . Nano Lett. 10 , 3692–3699 (2010).
Jaque, D. & Vetrone, F. Luminescence nanothermometry. Nanoscale 4 , 4301–4326 (2012).
Zhou, J. et al. Advances and challenges for fluorescence nanothermometry. Nat. Methods 17 , 967–980 (2020).
Smith, B. R. et al. Five-nanometer diamond with luminescent nitrogen-vacancy defect centers. Small 5 , 1649–1653 (2009).
Yuan, Y. et al. Biodistribution and fate of nanodiamonds in vivo. Diam. Relat. Mater. 18 , 95–100 (2009).
Wu, Y. et al. Diamond quantum devices in biology. Angew. Chem. Int. Ed. 55 , 6586–6598 (2016).
Fujiwara, M. et al. Real-time nanodiamond thermometry probing in vivo thermogenic responses. Sci. Adv. 6 , eaba9636 (2020).
Simpson, D. A. et al. Non-neurotoxic nanodiamond probes for intraneuronal temperature mapping. ACS Nano 11 , 12077–12086 (2017).
Choi, J. et al. Probing and manipulating embryogenesis via nanoscale thermometry and temperature control. Proc. Natl Acad. Sci. USA 117 , 14636–14641 (2020).
Jaque, D. et al. Nanoparticles for photothermal therapies. Nanoscale 6 , 9494–9530 (2014).
Lal, S., Clare, S. E. & Halas, N. J. Nanoshell-enabled photothermal cancer therapy: impending clinical impact. Acc. Chem. Res. 41 , 1842–1851 (2008).
Kamei, Y. et al. Infrared laser-mediated gene induction in targeted single cells in vivo. Nat. Methods 6 , 79–81 (2009).
Bath, D. E. et al. FlyMAD: rapid thermogenetic control of neuronal activity in freely walking Drosophila. Nat. Methods 11 , 756–762 (2014).
Hirsch, S. M. et al. FLIRT: fast local infrared thermogenetics for subcellular control of protein function. Nat. Methods 15 , 921–923 (2018).
Bastos, A. R. N. et al. Thermal properties of lipid bilayers determined using upconversion nanothermometry. Adv. Funct. Mater. 29 , 1905474 (2019).
Arai, S. et al. Mitochondria-targeted fluorescent thermometer monitors intracellular temperature gradient. Chem. Commun. 51 , 8044–8047 (2015).
Kiyonaka, S. et al. Genetically encoded fluorescent thermosensors visualize subcellular thermoregulation in living cells. Nat. Methods 10 , 1232–1238 (2013).
Baffou, G. et al. A critique of methods for temperature imaging in single cells. Nat. Methods 11 , 899–901 (2014).
Nagl, A., Hemelaar, S. R. & Schirhagl, R. Improving surface and defect center chemistry of fluorescent nanodiamonds for imaging purposes — a review. Anal. Bioanal. Chem. 407 , 7521–7536 (2015).
Wang, N. et al. Magnetic criticality enhanced hybrid nanodiamond thermometer under ambient conditions. Phys. Rev. X 8 , 011042 (2018).
Brookes, M. J. et al. Magnetoencephalography with optically pumped magnetometers (OPM-MEG): the next generation of functional neuroimaging. Trends Neurosci. 45 , 621–634 (2022).
Download references
Acknowledgements
This work was supported by the Moore Foundation. N.A. acknowledges support from the Alexander von Humboldt Foundation.
Author information
Authors and affiliations.
Department of Physics, Harvard University, Cambridge, MA, USA
Nabeel Aslam, Hengyun Zhou, Elana K. Urbach, Mikhail D. Lukin & Hongkun Park
Department of Chemistry and Chemical Biology, Harvard University, Cambridge, MA, USA
Nabeel Aslam & Hongkun Park
Institute of Condensed Matter Physics, Technische Universität Braunschweig, Braunschweig, Germany
Nabeel Aslam
Quantum Technology Center, University of Maryland, College Park, MD, USA
Matthew J. Turner & Ronald L. Walsworth
Department of Electrical and Computer Engineering, University of Maryland, College Park, MD, USA
Department of Physics, University of Maryland, College Park, MD, USA
Ronald L. Walsworth
You can also search for this author in PubMed Google Scholar
Contributions
All authors have read, discussed and contributed to the writing of the manuscript.
Corresponding author
Correspondence to Hongkun Park .
Ethics declarations
Competing interests.
The authors declare the following competing interests: R.L.W., M.D.L. and H.P. are scientific co-founders of QDTI Inc.
Additional information
Publisher’s note Springer Nature remains neutral with regard to jurisdictional claims in published maps and institutional affiliations.
Rights and permissions
Springer Nature or its licensor (e.g. a society or other partner) holds exclusive rights to this article under a publishing agreement with the author(s) or other rightsholder(s); author self-archiving of the accepted manuscript version of this article is solely governed by the terms of such publishing agreement and applicable law.
Reprints and permissions
About this article
Cite this article.
Aslam, N., Zhou, H., Urbach, E.K. et al. Quantum sensors for biomedical applications. Nat Rev Phys 5 , 157–169 (2023). https://doi.org/10.1038/s42254-023-00558-3
Download citation
Accepted : 10 January 2023
Published : 03 February 2023
Issue Date : March 2023
DOI : https://doi.org/10.1038/s42254-023-00558-3
Share this article
Anyone you share the following link with will be able to read this content:
Sorry, a shareable link is not currently available for this article.
Provided by the Springer Nature SharedIt content-sharing initiative
This article is cited by
Quantum sensing of micrornas with nitrogen-vacancy centers in diamond.
- Justas Zalieckas
- Martin M. Greve
- Riccardo Nifosì
Communications Chemistry (2024)
Quantum computing for oncology
- Siddhi Ramesh
- Teague Tomesh
- Alexander T. Pearson
Nature Cancer (2024)
Long-baseline quantum sensor network as dark matter haloscope
- Taizhou Hong
- Jiangfeng Du
Nature Communications (2024)
Quantum sensing for particle physics
- Steven D. Bass
- Michael Doser
Nature Reviews Physics (2024)
Titanium:sapphire-on-insulator integrated lasers and amplifiers
- Joshua Yang
- Kasper Van Gasse
- Jelena Vučković
Nature (2024)
Quick links
- Explore articles by subject
- Guide to authors
- Editorial policies
Sign up for the Nature Briefing newsletter — what matters in science, free to your inbox daily.


An official website of the United States government
The .gov means it’s official. Federal government websites often end in .gov or .mil. Before sharing sensitive information, make sure you’re on a federal government site.
The site is secure. The https:// ensures that you are connecting to the official website and that any information you provide is encrypted and transmitted securely.
- Publications
- Account settings
Preview improvements coming to the PMC website in October 2024. Learn More or Try it out now .
- Advanced Search
- Journal List
- v.6(14); 2020 Apr
Quantum biology revisited
Jianshu cao.
1 Department of Chemistry, Massachusetts Institute of Technology, 77 Massachusetts Avenue, Cambridge, MA 02139, USA.
Richard J. Cogdell
2 Institute of Molecular, Cell and Systems Biology, College of Medical, Veterinary and Life Science, University of Glasgow, Glasgow G12 8QQ, UK.
David F. Coker
3 Department of Chemistry, Boston University, 590 Commonwealth Avenue, Boston, MA 02215, USA.
Hong-Guang Duan
4 Atomically Resolved Dynamics Department, Max Planck Institute for the Structure and Dynamics of Matter, 22761 Hamburg, Germany.
5 I. Institut für Theoretische Physik, Universität Hamburg, Jungiusstrasse 9, 20355 Hamburg, Germany.
6 The Hamburg Centre for Ultrafast Imaging, Universität Hamburg, 22761 Hamburg, Germany.
Jürgen Hauer
7 Technische Universität München, Dynamische Spektroskopien, Fakultät für Chemie, Lichtenbergstr. 4, 85748 Garching, Germany, and Photonics Institute, TU Wien, 1040 Vienna, Austria.
Ulrich Kleinekathöfer
8 Department of Physics and Earth Science, Jacobs University Bremen, Campus Ring 1, 28759 Bremen, Germany.
Thomas L. C. Jansen
9 Zernike Institute for Advanced Materials, University of Groningen, Nijenborgh 4, 9747 AG Groningen, Netherlands.
Tomáš Mančal
10 Faculty of Mathematics and Physics, Charles University, Ke Karlovu 5, CZ-12116 Prague 2, Czech Republic.
R. J. Dwayne Miller
11 Departments of Chemistry and Physics, University of Toronto, Toronto, ON M5S 3H6, Canada.
Jennifer P. Ogilvie
12 Department of Physics, University of Michigan, Ann Arbor, MI 48108, USA.
Valentyn I. Prokhorenko
Thomas renger.
13 Institute of Theoretical Physics, Department of Theoretical Biophysics, Johannes Kepler University Linz, Altenberger Str. 69, 4040 Linz, Austria.
Howe-Siang Tan
14 Division of Chemistry and Biological Chemistry, School of Physical and Mathematical Sciences, Nanyang Technological University, 21 Nanyang Link, Singapore 637371, Singapore.
Roel Tempelaar
15 Department of Chemistry, Columbia University, 3000 Broadway, New York, NY 10027, USA.
Michael Thorwart
Erling thyrhaug, sebastian westenhoff.
16 Department of Chemistry and Molecular Biology, University of Gothenburg, Gothenburg 40530, Sweden.
Donatas Zigmantas
17 Chemical Physics, Box 124, Lund University, 22100 Lund, Sweden.
Associated Data
Supplementary material for this article is available at http://advances.sciencemag.org/cgi/content/full/6/14/eaaz4888/DC1
We revise the interpretation of coherence signals in photosynthetic systems and clarify what they tell us about light harvesting.
Photosynthesis is a highly optimized process from which valuable lessons can be learned about the operating principles in nature. Its primary steps involve energy transport operating near theoretical quantum limits in efficiency. Recently, extensive research was motivated by the hypothesis that nature used quantum coherences to direct energy transfer. This body of work, a cornerstone for the field of quantum biology, rests on the interpretation of small-amplitude oscillations in two-dimensional electronic spectra of photosynthetic complexes. This Review discusses recent work reexamining these claims and demonstrates that interexciton coherences are too short lived to have any functional significance in photosynthetic energy transfer. Instead, the observed long-lived coherences originate from impulsively excited vibrations, generally observed in femtosecond spectroscopy. These efforts, collectively, lead to a more detailed understanding of the quantum aspects of dissipation. Nature, rather than trying to avoid dissipation, exploits it via engineering of exciton-bath interaction to create efficient energy flow.
INTRODUCTION
Over the past decade, the field of quantum biology has seen an enormous increase in activity, with detailed studies of phenomena ranging from the primary processes in vision and photosynthesis to avian navigation ( 1 , 2 ). In principle, the study of quantum effects in complex biological systems has a history stretching back to the early years of quantum mechanics ( 3 ); however, only recently has it truly taken center stage as a scientifically testable concept. While the overall discussion has wide-ranging ramifications, for the purposes of this Review, we will focus on the subfield where the debate is most amenable to direct experimental tests of purported quantum effects—photosynthetic light harvesting.
In femtosecond multidimensional spectroscopy of several pigment-protein complexes (PPCs), we find what has been widely considered the experimental signature of nontrivial quantum effects in light harvesting: oscillatory signals—the spectroscopic characteristic of “quantum coherence.” These signals, or rather their interpretation with the associated claims of a direct link to the system’s “quantumness” ( 4 ), have drawn enormous attention, much of it from scientists outside the immediate community of photosynthetic light harvesting ( 5 ). While significant efforts have been spent on interpreting these weak signals, the overall debate has raised important questions of a general nature ( 6 ). What is uniquely “quantum” in biology? What “nontrivial quantum effects” can be considered as the origin of observable biological phenomena?
While addressing these questions has been extremely productive in terms of stimulating experimental and theoretical work, it has seemingly moved the discussion in photosynthesis away from actual biological function. The strong focus on coherence, specifically, has then led to a distorted view of natural photosynthesis. We identify two underlying assumptions in recent discussions: First, it occurs in both specialist and nonspecialist literature that coherence and quantumness are taken as equivalent terms and crucial to photosynthetic function (see the “Theoretical considerations: Coherence and quantumness” section for further discussion). Second, the narrow focus on the initial femtosecond dynamics draws attention away from the fact that light harvesting is, to a large extent, ruled by processes on time scales of tens of picoseconds ( 7 – 9 ). Thus, the efficiency bottlenecks are not found in the subpicosecond intraprotein relaxation, but rather in the orders-of-magnitude slower processes, such as intercomplex energy transfer and subsequent energy transduction steps in the form of electron transfer at the reaction center as discussed in the “Collective excitations and energy migration in light-harvesting systems” section below ( 10 , 11 ).
While it is crucial that rate-limiting processes are kept in mind, the main goal of this Review is to critically assess the persistence and role of quantum coherence in photosynthetic light harvesting. In more general terms, we believe that there is a deep understanding to be gained in tackling the emergence of the essentially classical world of biology from its quantized molecular origins. To collectively make progress in this interdisciplinary field, however, we find that having well-defined terminology and transparent definitions of fundamental concepts is of great importance. In this regard, we outline here what we consider the most useful picture of photoexcitation and energy migration in multipigment systems such as PPCs. We will then clarify the terms “coherence” and quantumness in the context of ultrafast spectroscopy of molecular systems, meanwhile posing suggestions for a transparent use of these terms. Following these definitions [see also ( 6 )], we analyze recent work on coherence in PPCs. Although we believe that our observations here generalize to a wide range of PPCs, we pay special attention to the Fenna-Matthews-Olson (FMO) protein, a light-harvesting complex from green sulfur bacteria, which has taken on an exemplary role in quantum biology.
Collective excitations and energy migration in light-harvesting systems
In essence, photosynthetic antennae are collections of pigments, such as (bacterio)chlorophylls and carotenoids, usually held in close proximity by a protein scaffold. The coupling between the pigments results in redistribution of transition energies and oscillator strengths, and when interacting with light, the pigments can no longer act as independent units. Because of this correlation between pigments, it is customary to describe transport within PPCs in terms of collective excitations—called “excitons” when vibrational-electronic mixing is weak—whose wave functions depend on the specifics of the coupling but generally extend over more than one pigment ( 12 ).
While the spectral observables of PPCs can be calculated in any basis of quantum mechanical states—for example, using the individual pigments (site basis) or otherwise—an excitonic description is desirable, because excitons represent the stationary eigenstates of the system. It is the signals from these states that are observed, e.g., in an optical absorption spectrum, and they are distinctly different from those associated with the isolated pigments.
In Fig. 1 , we depict how the tuning of pigment energies and their coupling result in the formation of delocalized excitons, whose spatial structure is used to direct energy transfer in the FMO complex. This excitonic level-to-level transfer has recently been fully mapped out ( 13 ) with the help of two-dimensional electronic spectroscopy (2DES) ( 14 ). More specifically, electrostatic interactions with the protein and solvent environment tune local pigment excitation energies (termed site energies) ( 15 – 18 ), and interaction between these energetically varying local states results in a ladder of excitonic states, where the higher energy states are localized toward the peripheral antenna complexes, while lower energy excitons are close to the photosynthetic reaction center ( 19 – 21 ). The protein and solvent environment not only act to tune the energy of the collective excitations but also play an essential function as the thermal bath into which excess energy can be dissipated. This efficient dissipation of excess energy, enabled by coupling between the excitons and vibrations ( 21 – 25 ), is crucial for fast and efficient energy transfer among the excitonic states.

( A ) Illustration of the excitation energy transfer in the FMO protein of green sulfur bacteria. The eight BChl a pigments of the monomeric subunit of the trimeric FMO protein are oriented as depicted. The excitation energy enters from the baseplate at the top and is transferred to the reaction center complex at the bottom. The blue, green, and red surroundings of the pigments indicate high-, intermediate-, and low-energy exciton states, respectively, to which the respective pigments contribute, as analyzed in detail in (D). ( B ) Time-dependent population of the exciton states [same color code as in (A)], assuming that the initial state is created by incoherent exciton transfer from the baseplate (section S5). ( C ) Time-dependent populations of local excited states, illustrated at four different times by illuminating the pigments accordingly. In addition, the exciton states are included as surroundings of pigments that appear and fade away according to the populations of these states in (B). ( D ) Analysis of the spatial extent of the different exciton states, using the density of exciton states d M (ω), eq. S20, shown in the top part, where the same color code is used for the different exciton states as in (A) to (D) and the exciton states pigment distribution functions d m (ω), eq. S21, shown in the lower three parts. ( E ) Interexciton coherences (left part) and their damping function (right part). ( F ) Damping functions of the optical coherences. In both (E) and (F), the coherences are initiated by assuming a δ-pulse excitation at time zero, and the quantum mechanical treatment of nuclear motion (red lines; eqs. S26, S27, S30, and S31) is compared with a classical treatment; see eqs. S33, S34, and S37, black lines. These calculations, as well as the calculations of the population transfer (B and C) were carried out for room temperature (300 K). The lower parts of figures S4, S6, and S8 show the population transfer obtained for a classical treatment of the nuclear motion, which fails to thermalize correctly. Two movies illustrating the spatial energy transfer as in (C) are available in the Supplementary Materials.
We must emphasize here that the warm, wet, and disordered environment of pigments in biological systems is far from the situation found in strongly coupled highly ordered solid-state systems, where excitations can be delocalized over the whole crystal. The interpigment coupling strength is often on the same order of magnitude as the interaction with the environment (bath), which, in combination with static disorder, results in a tendency to localize the excitation over a small number of pigments even in strongly coupled antenna complexes (e.g., order three to five pigments for LH1 and LH2) ( 26 , 27 ).
Theoretical considerations: Coherence and quantumness
In the recent literature, there are extended discussions of quantumness of energy transfer and its importance, e.g., for the robustness or efficiency of photosynthetic processes. It is increasingly common to see an equivalence being made between coherence and “nontrivial” quantum effects. However, coherence is not at all uniquely quantum but is also a well-known property of classical systems, e.g., for the motion of a pendulum or the propagation of electromagnetic waves ( 28 , 29 ), in which a well-defined phase relationship is maintained. As the existence of coherence by itself does not imply quantumness, its use as a descriptive term in discussions of “quantum coherent energy transport” calls for specification ( 6 ). However, the precise meaning of the term coherence is often left ambiguous, resulting in difficulty in discerning exactly what underlying physical phenomena are being discussed. In the interest of clarity, we provide a functional definition of coherence in the context of the observables in ultrafast spectroscopy as described in the following ( 6 ). It is interesting to realize that the issue of the relationships and subtle differences between coherences, “correlations,” and “intermolecular couplings,” as well as fundamental issues about how to treat the thermal equilibration of a quantum subsystem coupled to an environment (see below) also arose in great detail in the development of nuclear magnetic resonance a few decades ago, while considering coherent superpositions of spins on molecules separated by micrometers or millimeters in solution ( 30 , 31 ). Moreover, it had been clarified that a classical bath does not lead to a proper thermalization at low temperature ( 30 , 31 ).
Technically, the term coherence is used to denote off-diagonal elements in any density matrix. As the physical meaning of these off-diagonal matrix elements is completely dependent on the choice of basis (e.g., site or excitonic basis), however, we find this general definition too broad to be useful. Here, we thus prefer a more restrictive terminology, which essentially corresponds to the common use in recent ultrafast spectroscopy literature.
This problem of the definition of terms is well illustrated by the difficulties in communication between the experimental and theoretical communities. Specifically, we mean the difficulties arising when coherence is introduced as off-diagonal elements of the density matrix in the basis of localized pigment states. These coherences “appear” as oscillations in the site basis in theoretical simulations (fig. S5 shows an example of strongly damped oscillations in site basis coherences); however, the physical interpretation is simply that there is some degree of spatial delocalization of the excitations in the system ( 6 , 32 ). This information is useful when thinking about spatial relationships and degrees of localization of the excitons. However, there are only time-dependent (oscillatory) spectroscopic signals associated with these coherences whenever it is possible to selectively photoexcite isolated pigments in a coupled system. While careful tailoring of the laser pulse amplitude and phase may allow one (for a small number of systems) to create the necessary linear superposition of system eigenstates to achieve this, this approach is obviously confined to highly specialized laboratory settings.
We find a more useful definition of coherence, and the one most closely associated with the meaning of the term in recent experimental literature, to be the off-diagonal elements in the density matrix in the basis of system eigenstates (i.e., corresponding to the excitonic basis in the absence of vibronic mixing). The physical interpretation of these elements is a measure of the degree to which the (light-induced) state of the molecular system corresponds to a linear superposition of different eigenstates (e.g., excitons) of the system. These superpositions, when excited by short laser pulses, are nonstationary, evolving in time as damped oscillations with a frequency corresponding to the energy difference between the involved eigenstates.
In the context of dynamics, it is useful to make a further distinction: We refer to the specific superposition of ground and excited states as optical coherences. The evolution of optical coherence determines the transition frequencies and homogeneous linewidth of the absorption spectrum. In optical 2D experiments, it appears during the coherence times t 1 and t 3 (see Figs. 2 and and3 3 for details). Optical coherence provides information on the system-bath interactions relevant to electronic decoherence but has no simple relationship to energy transfer, which is a meaningful concept in the site basis. However, the two are not completely unrelated either, as our analytical estimate below will show.

( A ) In 2DES ( 14 ), a sequence of three laser pulses interacts with the sample, causing emission of a signal that is recorded as a function of the three time delays. For a given “population time” t 2 , the Fourier transform of the signal with respect to the t 1 and t 3 delays provides the respective excitation and detection frequency axes of the 2D spectrum. ( B ) Simple quantum two-level model for the energy levels of a photosynthetic pigment, giving rise to an inhomogeneously broadened absorption spectrum. In photosynthetic complexes, the protein environment tunes the electronic energy gap, and small conformational differences among proteins probed in an ensemble measurement cause shifts of energy gaps, broadening the absorption from its inherent homogeneous width. ( C ) A 2D spectrum recorded at t 2 = 0 separates homogeneous and inhomogeneous broadening, which are manifest as the antidiagonal and diagonal widths, respectively. ( D ) At later times ( t 2 > 0), dynamical interactions between the pigment and protein environment lead to energy gap fluctuations that broaden the antidiagonal width in a process termed spectral diffusion. The 2D spectrum contains both absorptive and refractive responses of the system; however, usually only the real part of the 2D spectrum is presented, corresponding to the absorptive part.

2D spectra have rich information about electronic structure and dynamics ( 14 ). Photosynthetic complexes contain light-absorbing pigments that are held in place by a protein scaffold that controls their relative distance and orientation, determining their coupling. In ( A ), we consider a common case of two weakly coupled pigments a and b. ( B ) At t 2 = 0, the 2D spectrum displays peaks along the diagonal that reveal the inhomogeneously broadened peaks at ω a and ω b , corresponding to absorption by pigments a and b, respectively. ( C ) At later times, if pigments a and b are sufficiently close in space and favorably oriented, then energy transfer may occur between them, with higher probability that energy flows “downhill” from the higher energy state of pigment b to pigment a. The energy transfer process leads to the formation of a cross-peak in the 2D spectrum. Recording 2D spectra as a function of population time t 2 enables the mapping of energy transfer pathways and time scales. In ( D ), we consider the case of two strongly coupled photosynthetic pigments. The strong coupling mixes the energy levels of the individual pigments, leading to excitons in which excitations are delocalized across the coupled pigments. Excitonic coupling between transitions is revealed by cross-peaks in the t 2 = 0, so-called correlation spectrum. Besides the population relaxation between two excitonic states, observed as growing of the lower cross peak, coherence is manifest as t 2 -dependent oscillations as shown in ( E ). The distribution of the oscillating signals on 2D maps can provide important insight into the physical origin of the coherence as discussed in Fig. 4 .
The coherences interpreted to be directly related to energy transfer correspond to superpositions of different excited states. These superpositions are nonstationary and evolve during the population time t 2 , e.g., between the pump and probe pulses in a transient absorption experiment. While superpositions between any set of system eigenstates (e.g., excitonic and vibronic) can be generated with the appropriate optical pulses, in particular, superpositions of excitonic states have been considered as important in the context of energy transfer. In the literature, these have been referred to as electronic or excitonic coherences. Here, we will refer to these specific coherences as interexciton coherences. Just as optical coherences, they evolve as damped oscillations in accordance with the energy differences between the involved system eigenstates. A number of factors, including the strength of the interaction with the bath, the exciton wave function overlap, and the lifetime of the involved states, determine the dephasing time of these interexciton coherences. As the strength of coupling to the bath influences the dephasing of both optical and interexcitonic coherences, fast-decaying optical coherence (observed as broad homogeneous line shapes) also indicates fast interexcitonic coherence decay. The specific relationship to make this connection depends on the degree of exciton localization and the bath dynamics and is detailed below.
The brief summary above provides what we consider a practical and useful definition of coherence as used in ultrafast spectroscopy studies. Note that these observations are valid both for classical and quantum representations, and the association of coherence in PPCs with classical oscillators turns out to be remarkably accurate (vide infra). The question remains, however: Where do we find quantumness in biology? Obviously, at the length scale of atoms, the world is governed by the wave properties of matter. The classical observables of biology are only concepts derived from this “true” quantum reality ( 33 ). But what truly quantum effects remain prominent in the classical, macroscopic world of biological systems, where much of the quantum mechanical “strangeness” is erased?
This question can be addressed by simplifying complex calculations and developing analytical theory to provide physical insight into quantum and classical aspects of the problem. The approach requires making controlled approximations to quantum complexity by introducing classical or semiclassical treatments in such a way as to preserve the fundamental bedrock of reality. These approximation methods can then be used to address the question of what quantum characteristics are essential to explain a particular phenomenon. A useful approach partitions the degrees of freedom of the full problem into those of the system of interest, and the rest involved in describing its surroundings, or the bath. The latter is typically formed by the protein scaffold and the solvent, which hosts the entire complex. There is a long history of mixing different types of models for these subsystems together with classical ( 34 ), quasiclassical ( 35 ), semiclassical ( 36 – 39 ), and fully quantum mechanical ( 40 – 45 ) descriptions of their dynamics, particularly in the context of how the spectrum of the system, and its relaxation and dissipation after excitation, can be influenced by the environment ( 46 , 47 ). Such a mapping can even be made exact ( 37 , 48 ). In the context of light harvesting, it has become apparent that electronic excitation may be described entirely by classical models ( 49 ) and that the coherent and incoherent regimes are common for both quantum and classical descriptions of their dynamics ( 50 ). Furthermore, it has been shown ( 29 , 47 , 51 ) that the excitons in PPCs can be treated as a set of classical oscillators, so even here, the strictly quantum nature of biology appears hidden. With the above qualification about mixing quantum and classical descriptions of the dynamics, it may be counterintuitive that a quantum description is most useful for the description of the bath dynamics—and in the coupling between the system and this bath ( 29 , 52 ).
To capture the relevant dynamics, we built the simplest approach that yields an analytical theory (outlined in the Supplementary Materials) on an approximate quantum dynamical description of the system interacting with a classical dynamical model for the bath. This approach gives an analytic result, and we shall use it for illustrative purposes. The critical observation in this quantum system/classical bath description of the dynamics is that it fails to capture thermalization of the system ( 29 , 52 – 54 ). In simple words, in a world where the behavior of electrons is governed by the fundamental equations of quantum mechanics and that of the nuclei by classical physics, there would be no preferential “downhill” energy flow, strongly impairing the macroscopic function of these complexes (figs. S4, S6, and S8). This relaxation process by coupling to bath modes is ultimately responsible for the directed transport of excitation energy ( Fig. 1, A and C ), the central function of these light-harvesting complexes. In contrast to the population of exciton states, which fail to correctly thermalize, the interexciton coherences can be well described by using a classical description of nuclear motion ( Fig. 1E ). Both the mixed quantum-classical and fully quantum descriptions agree that dephasing of interexciton coherences typically results in sub–100-fs decay times at room temperature. This is substantially shorter than intercomplex energy transfer times and hence cannot play any functional role.
Since the short lifetime of interexciton coherences is in the focus of this Review, in the following, we provide its theoretical foundation and possible functional implication with the above considerations by properly treating the bath interaction. The good agreement between the fully quantum mechanical and the mixed quantum-classical damping of interexciton coherences, discussed above, allows us to use the simple mixed quantum-classical expression, derived in the Supplementary Materials (sections S4 and S6), to reliably capture the general behavior. In this classical environment limit and within the Frenkel exciton model, the decay of interexciton coherence ρ MN between the M th and N th exciton states is given as Γ MN ( t ) = exp ( − ( τ M − 1 + τ N − 1 ) t ) exp ( − κ MN E λ k B T ℏ 2 t 2 ) , where the first factor contains the dephasing constants τ M and τ N that equal twice the lifetimes of the exciton states ∣ M 〉 and ∣ N 〉, which are determined by exciton relaxation (eq. S18). In the second factor, termed pure dephasing, E λ is the reorganization energy of the pigment’s local transition energy fluctuations, and κ MN = ∑ m ( ∣ c m ( M ) ∣ 2 − ∣ c m ( N ) ∣ 2 ) 2 contains the probabilities ∣ c m ( M ) ∣ 2 and ∣ c m ( N ) ∣ 2 of the m th pigment being excited in exciton states ∣ M 〉 and ∣ N 〉. The inverse of κ MN is a measure for the intrinsic correlation in the fluctuations of the M th and N th exciton energies. For any two excited states, which delocalize exactly the same way over the same pigments, κ MN becomes zero, and the correlation is perfect. For the optical coherence ρ M 0 (0 denotes the global ground state), the damping function reduces to Γ M 0 ( t ) = exp ( − t / τ M ) exp ( − Λ M E λ k B T ℏ 2 t 2 ) , where the inverse participation ratio Λ M = ∑ m ∣ c m ( M ) ∣ 4 quantifies the delocalization of the M th exciton state. In the limit of localized electronic states, we have Λ M = 1 and κ MN = 2, there is no lifetime dephasing ( τ M − 1 = 0 ; see eq. S18, because of zero spatial overlap between excited states). In this case, the interexciton coherences decay faster than the optical coherences. For completely delocalized excited states, κ MN becomes zero, and we have Λ M = 1/ N P , with the number of pigments N p . Thus, the pure dephasing does not affect interexciton coherences, whereas its effect on optical coherences is suppressed. In this case, it is likely that the lifetime dephasing, which is enhanced for strong spatial overlap of exciton states (eq. S19), will dominate the dephasing of both types of coherences. If so, there is a general trend that the interexciton coherences for delocalized states (and within the Frenkel exciton model) will also decay faster than the optical coherences. We note that these arguments rely on several approximations, most notably, a secular approximation (justified for the FMO complex in fig. S2), which we use here to illustrate the central issue of coherence lifetimes. To achieve a more accurate description of reality, more refined and numerically exact tools might have to be used ( 42 , 55 – 58 ). In addition, alternative quantifiers of quantumness on the basis of energy current operators can be applied ( 42 ).
For the FMO protein, the calculated dephasing times of interexciton and optical coherences are in the range of 50 and 75 fs, respectively ( Fig. 1, E and F ), significantly shorter than the lifetimes of the exciton states ( Fig. 1B ), showing the dominance of pure dephasing processes. This result reflects the partial localization of excited states and their modest spatial overlap in this system ( Fig. 1D ).
With the expected extremely fast decoherence, it is appropriate to consider an alternative mechanism (beyond the spatial overlap of excitons) that could lead to long-lived interexciton coherences: correlations in site energy fluctuations of different pigments. This correlation has not been included in the theoretical considerations above. A number of studies have advocated such “environmental protection of excitonic coherence” as the source of long-lived oscillations in 2D spectra ( 59 – 61 ). However, no quantum mechanics/molecular mechanics based dynamic studies of the FMO protein could identify correlations in site energy fluctuations ( 22 , 25 ). In a normal mode analysis of the FMO spectral density, correlations were found but only at very low vibrational frequencies ( 23 ). These correlations were calculated to have practically no influence on the populations of exciton states. Moreover, while artificially introducing these correlations for higher-frequency components of the spectral density can lead to protection of interexciton coherences, they will, at the same time, markedly hamper exciton relaxation and, thereby, the spatial transfer of excitation energy. Hence, it has to be concluded that correlations in site energy fluctuations, which would allow for long-lived interexciton coherences are detrimental for the light-harvesting function ( 23 ). Whether coherence is actually generated under natural excitation conditions (i.e., by sunlight) is still a heavily debated topic ( 62 – 64 ), with some works dismissing the idea of coherence under sunlight ( 62 , 63 ), while others follow an early suggestion of representing sunlight by a series of ultrashort bursts ( 65 ). We would like to point out that, in a secular approximation (justified for the FMO protein; see fig. S2), the evolution of interexciton coherences is independent of the evolution of populations, obviously excluding a direct functional influence of these coherences.
From a structural point of view, it is the inhomogeneous charge distribution in the FMO protein that, on one hand, leads to varying site energies of the pigments and, on the other hand, gives rise to different local exciton-vibrational coupling constants, suppressing correlations in site energy fluctuations. The first effect is used to direct the excitation energy toward the reaction center, the second effect leads to an efficient dissipation of the excess energy of excitons. Both lead to the observation of a fast decay of interexciton coherences in femtosecond spectroscopy experiments. As the electrostatic tuning of site energies by the protein environment is used by many photosynthetic PPCs, e.g., those of higher plants ( 9 , 66 ), we think that the mechanisms analyzed above for the FMO protein are quite general.
In the following, we will investigate how coherence manifests itself in experimental spectra—oscillatory features in specific spectral regions—and critically evaluate the interpretation of these experimental observables for the representative FMO case. We will point out that the interpretation of these oscillations as originating from superpositions of exciton states (rather than from vibrations) is incorrect and needs to be revised on the basis of several experimental and theoretical studies ( 39 , 67 – 69 ).
Experimental considerations: Coherence in ultrafast spectroscopy
When experimentally addressing nonstationary coherences, it is important to understand how these coherences, appearing as oscillating signals and referred to here as “quantum beats” (QBs), are excited. Fundamentally, observation of QBs requires a laser spectrum broad enough to cover transitions of all the states involved in the coherence, in other words, the laser must contain the resonance frequencies of all involved oscillators. In addition, the laser pulses have to be equal or shorter than the period of the QBs to provide the required time resolution. As laser excitation creates superpositions of any states with allowed transition dipole moments, care has to be exercised to distinguish coherences associated with excitons. For example, if a laser spectrum covers two states in a vibrational progression, either in the ground or in the excited electronic state, the induced signal is due to concerted motion of a nuclear mode in the molecules, i.e., the observation of vibrational coherence. Conversely, in the case of two electronic (or exciton) states, a well-defined phase relation will be initiated—electronic (or interexcitonic) coherence ( 70 ). In between these limiting cases arises the general situation of superpositions of states with mixed vibrational-electronic character, which defines vibronic coherence—a field of significant current interest ( 71 ). As both electronic ( 72 ) and purely vibrational coherences ( 73 – 75 ) modulate ultrafast spectra in the form of periodic oscillations, the need to distinguish between them is obvious. As discussed previously ( 76 , 77 ), the assignment of long-lived small-amplitude QBs in several photosynthetic systems to interexciton coherences based solely on their frequencies and sometimes phase ( 78 – 80 ) is insufficient.
Further complications in assignment arise because of disorder and spectral congestion. 2DES (see Figs. 2 and and3) 3 ) was initially introduced to remove inhomogeneous broadening with the hope to directly observe interexciton couplings and fully resolve the energy transfer pathways ( 81 , 82 ). However, this hope has not been generally realized. Severe spectral congestion for multipigment systems often leads to overlap of oscillatory signals, with strongly distorted features due to interference effects. Given the number of possible spectral features in PPCs, their assignment is far from trivial even in the well-resolved 2DES experiment.
Studies of photosynthetic excitons
We reiterate that the core of the initial argument for significant involvement of interexciton coherence—or any coherence—in photosynthetic systems was the observation of long-lived oscillations in electronic 2D spectra ( 4 ). These were interpreted to originate from linear superpositions of excitonic states, exhibiting dephasing times of several hundred femtoseconds or more. This was taken to imply a connection to energy transfer dynamics. While these experiments were fundamental to the development of quantum biology as a field, it is important to recognize that coherence dynamics has a much longer history than this recent explosion of interest might suggest. The first such observation was made already in 1991 by Vos et al . ( 83 ) in a low-temperature study of the purple bacteria reaction center. Here, however, the authors assigned the QBs to vibrational wave packet motion on the excited state ( 84 ). In another early study, Chachisvilis et al . ( 85 ) came to a similar conclusion in their study of the core light-harvesting complexes (LH1 and LH2) of purple bacteria: The observed QBs were caused by vibrations. The first observation of interexciton coherences contributing to the signals of PPCs was made by Savikhin et al . ( 86 ), who, in 1997, observed oscillations in the pump-probe anisotropy of the FMO complex at 19 K. The fast dephasing of ~200 fs agreed well with naïve expectations for dephasing of interexciton coherence in a biological system at low temperatures and suggested that this dephasing of coherences in biological conditions should be too fast to contribute significantly to the light-harvesting function. It thus came as a substantial surprise when, a decade later, oscillatory signals persisting for >600 fs ( 4 ) in the same complex at 77 K were reported and assigned to long-lived interexciton coherences. Not only did this proposal imply that decoherence was much slower than allowed by a realistic physical model at the time but also that the extremely successful paradigm of energy transfer in light-harvesting systems, based on incoherent transport of energy between partially-localized exciton states, would have to be revised. Similar spectral signatures—small-amplitude oscillations—were reported in other organisms ( 79 , 80 , 87 , 88 ), leading to speculations that long-lived interexciton coherence was ubiquitous in natural photosynthesis, i.e., nature had discovered a design principle to exploit quantum coherences to direct biological functions.
While these studies have led to enormous interest in coherent phenomena, realistically, the experimental basis for the excitonic interpretation suffered from a selective view of the 2D spectra. In particular, for practical signal-to-noise reasons, these studies largely relied on kinetic traces extracted from only a very limited—typically one or two—areas of the 2D spectra. As we show in Fig. 4 , coherence signals are often complex and difficult to reliably identify ( 67 ), calling for a more holistic interpretation of the entire 2D dataset. In the presence of vibronic mixing of electronic and vibrational states—which seems to be the case in many photosynthetic complexes—coherence signals are very much entangled, and an oscillation map analysis ( Fig. 4 ) is indispensable ( 89 ). To outline a basic framework for the interpretation of QBs in 2D spectra and to provide readers with some basic tools to qualitatively assess 2D experiments on QBs, we schematically show their analysis in two relevant model systems in Fig. 4 .

Coherences of different physical origin—vibrational or excitonic—lead to different characteristic patterns in the so-called oscillation maps. The characteristics of these signals—such as frequency, pump dependence, and detection dependence—provide unique identifiers, at least in idealized systems. Comparing the complicated signals from these systems to model systems proves very helpful. In ( A ), simple and useful model systems are the displaced oscillator (top) featuring ground- and excited-state vibrations and the excitonic dimer (bottom), where excited-state splitting is induced by coupling between pigments. ( B ) Even these simple models may yield virtually indistinguishable 2D spectra, with coherence manifested as QBs in the signal amplitude at specific spectral coordinates. When following these beats along the population time t 2 , one can observe periodic modulations of the real (RE; absorption) and imaginary (IM; dispersion) parts of the signal (black/gray and green/light green, respectively). a.u., arbitrary units. ( C ) Successful assignment of oscillatory signals to physical phenomena requires simultaneous analysis of beats in the entire 2D map, yielding oscillation maps after complex Fourier transformation (FT). These oscillation maps are most insightful when retrieved separately for rephasing (photon-echo) and nonrephasing responses. We here sketch rephasing data of a system with three closely spaced, but distinguishable, coherences: an excited state vibrational wave packet (top left), a Raman-active ground-state vibrational mode (top right), and an excitonic coherence (bottom). The oscillation maps show unique patterns, allowing unambiguous identification of coherences.
Present status of experiments: Revisiting FMO
In the debate on the importance of quantum coherences in photosynthesis, the FMO complex has again taken center stage because of its exemplar status in quantum biology. Here, we outline several recent studies of this complex, each reaching the same conclusion: The observed long-lived QBs are inconsistent with interexciton coherence. Instead, these oscillations mostly show characteristics of Raman-active vibrational modes on the electronic ground-state surface, unrelated to the energy transfer process.
The collaborative work of the Miller, Thorwart, and Cogdell groups, Duan et al . ( 90 ), addressed the question of whether the oscillatory signals were observable on functionally relevant time scales at biologically relevant temperatures. In contrast to the earlier studies, mostly performed at cryogenic temperatures [with a notable study at ~4°C (277 K) ( 88 )], the authors found no low-frequency QBs with dephasing times beyond 60 fs. On the basis of the observed time scales for energy transfer within the exciton manifold, this work demonstrated that interexciton coherence cannot contribute meaningfully to energy transfer dynamics at physiological temperatures. The correct picture for energy transport involves incoherent relaxation between exciton states, downhill in energy. Since these exciton states are partially localized, this relaxation corresponds to a hopping between different spatial regions of the complex, giving the transport a direction ( Fig. 1 ).
The Scholes and Blankenship groups, Maiuri et al . ( 91 ), came to a similar conclusion in a study at cryogenic temperatures. They took an approach relying on the direct correspondence between the energy gap between excitonic levels and the frequency of the interexciton QB signal. By altering the energy gaps in the excitonic structure using genetic engineering, one would expect the frequency of the observed oscillation should change. In contrast, the authors’ observations for a series of transient absorption experiments on FMO mutants with radically different excitonic splittings were that the QB frequencies were essentially unchanged. While this mutation-based approach is difficult to implement as a general analysis strategy for all light harvesters, for FMO, it provided unambiguous evidence for vibrational rather than interexciton coherence.
Last, the Zigmantas, Knoester, and Jansen groups, Thyrhaug et al . ( 92 ), investigated the wild-type FMO complex at cryogenic temperatures using polarization-controlled 2DES. They relied on pattern analysis ( Fig. 4 ) to identify the QBs corresponding to specific types of coherences. This approach has been validated in studies on isolated bacteriochlorophyll a pigments ( 93 ). Again, the authors concluded that the long-lived QBs in the FMO protein were predominantly originating from Raman-active ground-state vibrations, with some contribution from excited state vibrational coherences. While electronic coherences were also identified, these were found ( 92 ) to be fully damped within 240 fs—in agreement with the earlier work of Savikhin et al . ( 86 ). Note that, through the use of polarization-controlled spectroscopy, it was possible to identify vibronically mixed excited states in the complex.
Individually and collectively, these studies demonstrate that the long-lived QBs in the FMO protein—one of the most heavily studied PPCs—are vibrational in origin. Thus, the interpretation of long-lived QBs in the FMO protein, as characteristics of interexciton coherence posed in 2007 ( 4 ) and in several subsequent studies ( 79 , 80 , 87 , 88 ), has been replaced by a well-founded vibrational picture.
The working paradigm of an energy gradient and spatial proximity guided incoherent exciton transport, rather than the “wave-like” dynamics implied by the interexciton coherence picture, has been reestablished as the framework of choice in photosynthetic light harvesting. Is this conclusion reached for the FMO complex generic? We believe, yes, as the general mechanism of variable site energies directing the energy automatically leads to partial localization of the exciton states, with spatially uncorrelated bath fluctuations over these sites. Correspondingly, the loss of interexciton coherence is rapid under ambient conditions. Other ubiquitous natural photoactive complexes such as the Light-Harvesting Complex II ( 94 ) or the reaction center of the Photosystem II ( 95 ) show similarly fast electronic decoherence rates.
We must note that the world of photosynthetic light harvesting still remains interesting, as recent work has shown that much more complicated dynamics may appear when vibrations and electronic energy gaps are in resonance. These resonances lead to inseparability of nuclear and electronic degrees of freedom ( 96 , 97 ), which some theoretical work has suggested may affect energy transfer ( 98 ). These resonances have also been reported in photosynthetic reaction centers ( 89 , 99 , 100 ). Yet, the character of a quantum state, which is initially strongly vibronic in nature, changes over time in a nontrivial way when the electronic and vibrational sectors are exposed to different dephasing and relaxation channels on very different time scales. The amplitude of the antiresonant vibrational mode of two coupled monomers can be enhanced by a strong coupling to a long-lived coherent electronic state when the latter evolves without ( 96 ) or under weak ( 101 ) electronic dephasing. However, the fast electronic dephasing, which appears to be general for light-harvesting systems, destroys coherence in the electronic sector faster than the vibrational period. Consequently, the vibrational coherence in the sector of the antiresonant vibrational mode, under realistic conditions, remains unaffected in this limit of short-lived electronic coherences ( 102 ). In this respect, we believe that considerable care must be taken in interpreting the resulting spectral signatures of vibronic coherences—and, in particular, when attempting to place this physics in the context of biological function. The rich spectroscopic information obtainable by 2DES should provide essential input for models of these complex situations, and these models will be an important next step for testing the putative functional significance of electronic-vibrational resonances for photosynthetic function. We hope that forthcoming studies will ultimately improve both our understanding of nature’s remarkable photosynthetic processes and our ability to mimic nature’s best “ideas” in artificial light-harvesting materials.
CONCLUSIONS
In summary, we have revisited the quantum aspects of photosynthetic light harvesting. It has become clear from basic considerations that there is no equivalence between quantumness of the processes and coherences observed in femtosecond spectroscopy experiments. Even the very fundamental question if nonstationary coherences in photosynthetic systems can be excited by sunlight still awaits full clarification ( 62 – 64 , 103 ). Whatever the state preparation is, the dynamics will be governed by the associated couplings of the system and its interaction with the bath. Furthermore, the claims of the persistence of these coherences in femtosecond experiments have been critically reevaluated. In particular, detailed analysis of the exemplar system in quantum biology—the FMO complex—shows unambiguously the absence of long-lived interexciton coherence on relevant time scales in this system, both at cryogenic and physiological temperatures. Instead, it has become clear that the long-lived oscillating signals originate from vibrational modes predominantly on the electronic ground state. More advanced data analysis and theoretical treatments using realistic parametrization of the bath are needed for clear identification of coherence signals. The extensive discussion of earlier assignment of these spectral signatures, propagating in the community for a decade, underlines this need.
The major positive outcome is the improvement of theoretical and experimental methods that have led to a deeper understanding of the system-bath interactions responsible for decoherence and dissipation in biological systems. Nature does not engineer the bath to avoid decoherence to direct functional processes; such an approach almost certainly would not be robust. Nature, rather than trying to avoid dissipation, specifically exploits it together with the engineering of site energies and excitonic coupling to direct energy transport. The role of thermodynamic parameters in driving biological functions is well appreciated on other levels. Here, we see that this principle applies even to the energy transfer processes involved in photosynthesis that occur on the fastest possible time scales. The basic physics behind thermalization is used to impose direction. This simple concept, mastered by nature over all relevant time and spatial dimensions, is truly a marvel of biology.
Time-local density matrix theory in the representation of exciton states (see the Supplementary Materials for details) is applied using a Markov and a secular approximation for the off-diagonal elements of the exciton-vibrational coupling ( 104 ) for the description of optical line shapes (section S3), the decay of optical and interexciton coherences (section S4), and exciton relaxation (sections S3 to S5). The parameters of the Frenkel exciton Hamiltonian of the FMO protein (section S2) are taken from a quantum chemical/electrostatic study ( 17 ) (site energies and excitonic couplings; see table S1) and from an analysis of fluorescence line-narrowing and temperature-dependent absorption spectra (spectral density of exciton-vibrational coupling) ( 105 ). These parameters, which are tested against linear optical spectra (fig. S3), are used in calculations of exciton relaxation initiated by incoherent transfer from the baseplate ( Fig. 1, B and C , section S5, and figs. S6 to S8) and in the calculations of the decay of interexciton ( Fig. 1E ) and optical ( Fig. 1F ) coherences initiated by a δ-pulse excitation (section S4). The classical limit of the nuclear motion (black lines in Fig. 1, E and F ; and figs. S4, S6, and S8) is obtained by solving Hamilton’s equations of motion for the nuclei and performing an average over Boltzmann-distributed initial coordinates and momenta in the calculation of the energy gap correlation function of the pigments, which enters the damping function of coherences and the rate constant (section S6).
Supplementary Material
Acknowledgments.
Funding: D.F.C. acknowledges the support of U.S. National Science Foundation (NSF) grant CHE-1665367. D.Z. acknowledges support from the Swedish Research Council. H.-G.D. acknowledges financial support by the Joachim-Herz-Stiftung Hamburg within a PIER fellowship. The work of H.-G.D. and R.J.D.M. was supported by the Max Planck Society. Moreover, H.-G.D., M.T., and R.J.D.M. were supported by the Cluster of Excellence “CUI: Advanced Imaging of Matter” of the Deutsche Forschungsgemeinschaft (DFG) - EXC 2056 - project ID 390715994. H.-S.T. acknowledges support from the Singapore Ministry of Education Academic Research Fund (Tier 2 MOE2015-T2-1-039). U.K. is grateful for a Tan Chin Tuan Exchange Fellowship for a research stay at Nanyang Technological University, Singapore. J.C. acknowledges funding through NSF CHE 1836913 and NSF CHE 1800301. J.H. acknowledges funding by the DFG (German Research Foundation) under Germany’s Excellence Strategy EXC 2089/1390776260. J.P.O. acknowledges support from the Office of Basic Energy Sciences, the U.S. Department of Energy under grant number DE-SC0016384, and the NSF under grant number PHY-1607570. R.J.C. gratefully acknowledges support from the Photosynthetic Antenna Research Center, an Energy Frontier Research Center funded by the U.S. Department of Energy, Office of Science, Office of Basic Energy Sciences under award number DE-SC 0001035. S.W. and D.Z. acknowledge support from the Knut and Alice Wallenberg Foundation. T.M. is supported by the Czech Science Foundation (GACR) grant 17-22160S. Author contributions: The authors met many times over the past 2 1 / 2 years, both in person at meetings and by videoconference to thoroughly discuss and debate the reviewed topics in the field to arrive at the consensus view presented in this paper. T.R. carried out the calculations presented in Fig. 1 and in the Supplementary Materials. All authors contributed to the planning, writing, and all stages of review of the manuscript. Competing interests: The authors declare that they have no competing interests. Data and materials availability: All data needed to evaluate the conclusions in the paper are present in the paper and/or the Supplementary Materials. Additional data related to this paper may be requested from the authors.
SUPPLEMENTARY MATERIALS
View/request a protocol for this paper from Bio-protocol .
REFERENCES AND NOTES

IMAGES
COMMENTS
Quantum biology is the study of such processes, and here we provide an outline of the current state of the field, as well as insights into future directions. 1. Introduction. Quantum mechanics is the fundamental theory that describes the properties of subatomic particles, atoms, molecules, molecular assemblies and possibly beyond.
Quantum biology studies span multiple disciplines including physics, engineering, and biology with the goal of understanding the quantum underpinnings of living systems. Recent findings have brought wide attention to the role of quantum mechanisms in ...
Recently, extensive research was motivated by the hypothesis that nature used quantum coherences to direct energy transfer. This body of work, a cornerstone for the field of quantum biology, rests on the interpretation of small-amplitude oscillations in two-dimensional electronic spectra of photosynthetic complexes.
Explore the latest full-text research PDFs, articles, conference papers, preprints and more on QUANTUM BIOLOGY. Find methods information, sources, references or conduct a literature review on ...
Research in this field might reveal new quantum mechanical principles for improving the efficiency of energy harvesting in biology. a , Diagram of the photosynthetic apparatus of green sulphur ...
Understanding the rules of life is one of the most important scientific endeavours and has revolutionised both biology and biotechnology. Remarkable advances in observation techniques allow us to investigate a broad range of complex and dynamic biological processes in which living systems could exploit quantum behaviour to enhance and regulate biological functions. Recent evidence suggests ...
Bearing in mind the limitations of existing quantum computing devices, we attempt to indicate promising directions for further research in the emerging area of quantum computational biology.
The recent advances of quantum biology suggest a potential role in biomedical research. Studies related to electromagnetic fields, proton pumping in mitochondrial respiratory chain, quantum theory of T-cell receptor (TCR)-degeneracy, theories on biophotons, pyrophosphates or tubulin as possible carriers for neural information, and quantum properties of ions and protons, might be useful for ...
Gerstein has a supplemental grant to explore quantum computing. At the moment, the areas he sees most amenable to quantum computing are calculations in drug design and structural biology.
This review aims to investigate the current state of this research and how fully the theory is supported by convincing experimental evidence. It also aims to clarify the biological sites of these proposed quantum effects and how progress made in the wider field of quantum biology might be relevant to the specific case of the brain.
The present Special Issue "Recent Advances in Quantum Biology" aims to collect and publish recent advances in the area of quantum biology. We welcome all reviews and research articles concerned with molecular level quantum phenomena observed in biological systems at functional, cellular, or organism levels.
The recent progress of quantum information science and nanotechnology, together with the increased interest in the role of quantum nonlocality in living systems (Arndt et al, 2009; Ball, 2011), provide fresh impetus to Quantum Biology, a field at the intersection of non-classical physics and biology. This paper explores some theoretical and experimental aspects of the research program we ...
The new research expands the role of quantum biology to more complex systems in which quantum dynamics might be enhanced, rather than washed away, by a finely tuned and constructive interplay between the quantum system and its surroundings.
Quantum biology challenges long-held beliefs that limit quantum effects to microscopic scales within the warm and wet conditions of life and pushes the boundaries of our understanding of biology by examining how quantum effects, once thought to be relevant only at the microscopic scale, can play a role in complex, macroscopic biological systems ...
Quantum Biology is emerging as a new field at the intersection between fundamental physics and biology, promising novel insights into the nature and origin of biological order. We discuss several elements of QBCL (Quantum Biology at Cellular Level), a research program designed to extend the reach of quantum concepts to higher than molecular levels of biological organization. Key words ...
Abstract: Understanding the rules of life is one of the most important scientific endeavours and has revolutionised both biology and biotechnology. Remarkable advances in observation tech-niques allow us to investigate a broad range of complex and dynamic biological processes in which living systems could exploit quantum behaviour to enhance and regulate biological functions. Recent evidence ...
Quantum biology is the application of quantum theory to aspects of biology for which classical physics fails to give an accurate description. In spite of this simple definition, there remains debate over the aims and role of the field in the scientific community.
Quantum computing uses the laws of quantum mechanics to perform computations. Quantum mechanics is the physical theory that governs all matter but is particularly relevant at the molecular scale ...
Understanding the possible quantum-driven behaviors of biological systems could aid in treating injuries or in developing cures for diseases, but research in the field has been pushed to the sidelines. It's time for that to change.
This paper presents a historical perspective on the development and application of quantum physics methodology beyond physics, especially in biology and in the area of consciousness studies.
A new paper on their research, co-authored by Srinivasa, Jacob M. Taylor of the University of Maryland and the National Institute of Standards and Technology, and Jason R. Petta of the University ...
The key to practical quantum computing and high-efficiency solar cells may lie in the messy green world outside the physics lab.
By characterizing sources of energy loss and optimizing quantum circuits, scientists have reached a major performance milestone for quantum devices.
Quantum sensors enable new possibilities in biomedical applications due to their high sensitivity. In this Review, the status of quantum sensing is presented, and the path towards real-world ...
Abstract. Photosynthesis is a highly optimized process from which valuable lessons can be learned about the operating principles in nature. Its primary steps involve energy transport operating near theoretical quantum limits in efficiency. Recently, extensive research was motivated by the hypothesis that nature used quantum coherences to direct ...