- Write my thesis
- Thesis writers
- Buy thesis papers
- Bachelor thesis
- Master's thesis
- Thesis editing services
- Thesis proofreading services
- Buy a thesis online
- Write my dissertation
- Dissertation proposal help
- Pay for dissertation
- Custom dissertation
- Dissertation help online
- Buy dissertation online
- Cheap dissertation
- Dissertation editing services
- Write my research paper
- Buy research paper online
- Pay for research paper
- Research paper help
- Order research paper
- Custom research paper
- Cheap research paper
- Research papers for sale
- Thesis subjects
- How It Works

What Is The Thesis Statement Of The Research Report Life On Mars?
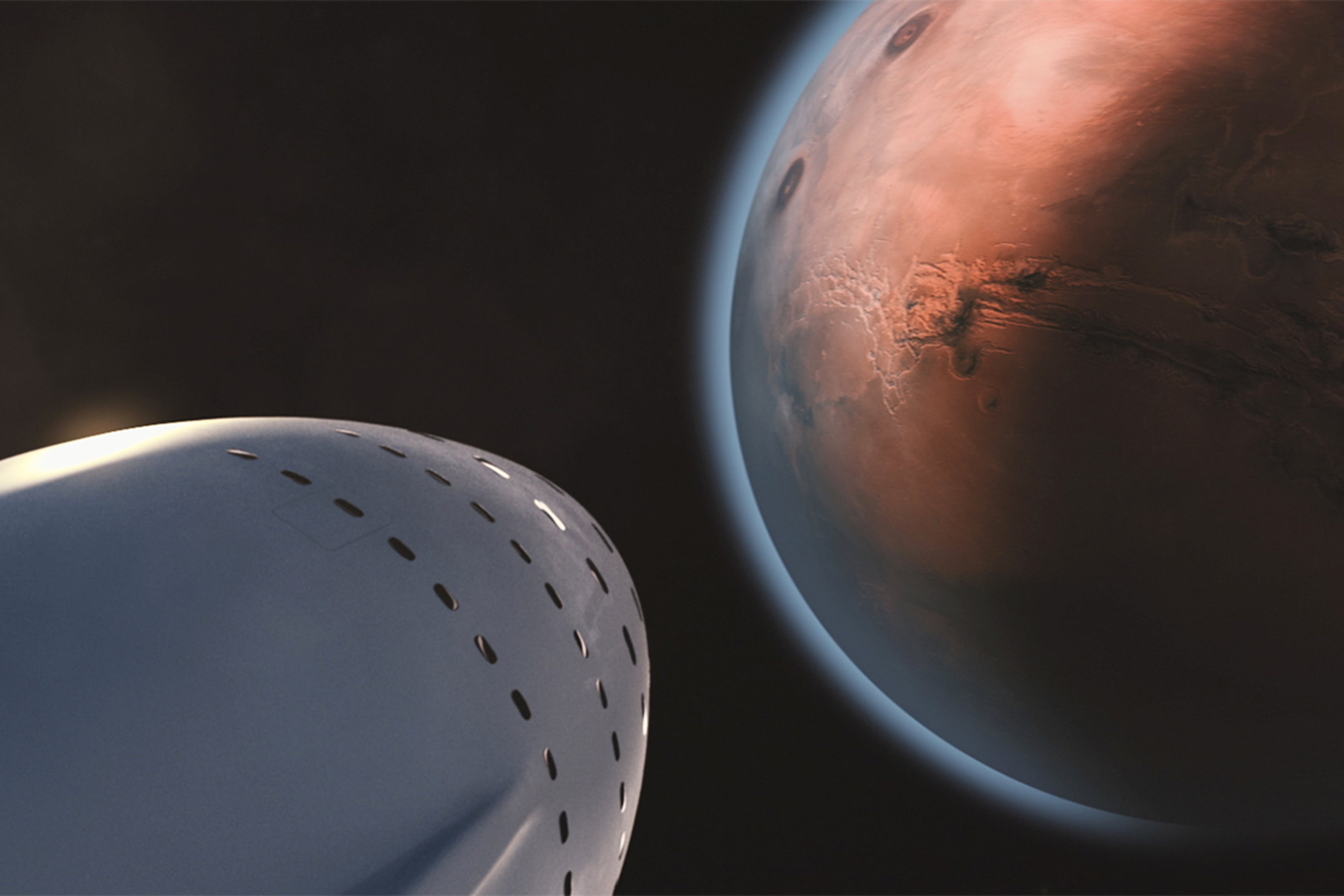
Many students look for an excellent way to start a research paper on life on mars. However, to write a good thesis statement on the topic, a student must understand what a thesis statement is and its importance. This article aims to enlighten you on how to write a great thesis statement and give you some suggestions on thesis statements ideas you can use for your upcoming essay. But first: a thesis statement appears in the introductory paragraph and presents the essay’s main topic. In your life on mars paper, the thesis statement should introduce the reader to what you’ll be talking about. Your thesis statement is the most crucial part of an essay because it also sets the tone for the rest of the paper.
What Can You Write About in Your Life on Mars Thesis?
How to start thesis statement of the research report life on mars, why introduction to research report on life on mars matters, topics to write in your life on mars research paper thesis statement, let’s help you write the best life on mars thesis statements and papers.
There are three approaches you can use to write a thesis statement about life on Mars. They include giving detailed explanations, arguing your facts, and analyzing the topic. Here’s more to that:
- Explanatory thesis statement: This is where the writer gives a detailed account of a particular topic on life on mars. It requires you to be accurate on facts you write down and present evidence. These thesis statements do not support or oppose any claim.
- Argumentative thesis statement: Such a thesis statement requires you to express a specific view on life on Mars. After that, back or oppose your idea while supporting your stand. You need plenty of research to back your opinion. An example is ‘There can be no life on Mars.’
- Analytical thesis statement: It requires analysis of a particular topic, defining it, noting its various features, and evaluating it. Such thesis statements are suitable for writing papers where data analysis dominates.
An introduction to a research report done correctly will help you structure your argumentative, analytical or explanatory essay . Here are the steps to follow when writing your thesis statement on the research report on life on Mars:
- Conceptualize your thesis statement. It’s always a good idea to brainstorm and have the statement ready before writing. Have the relevant research to help you develop the body of your essay. In this case, read about life on Mars from several materials to establish points to write about.
- Answer a certain question. Your thesis statement should be an answer to a specific question. It should inform the reader what you’ll be writing about. For instance, a thesis statement such as ‘How life on mars negatively affects human life’ tells the reader that you intend to write about the adverse effects of mars on human life.
- Go straight to the point. A good thesis statement should be brief. While a couple of sentences may be acceptable, you should express your point in one sentence. For instance, an ideal thesis statement would be’ Human life on Mars is not possible.’
The introduction of any research report informs the reader of the paper’s contents. It gives some information on the subject that will be discussed. An excellent example of an introduction ought to:
- Provide the reader with background information about Mars.
- Talk about features of Mars similar to other planets like Earth.
- Briefly explain what you intend to write.
Are you figuring out what to write? The following are some examples regarding life on Mars that you can use as inspiration in your paper:
- How life on Mars would positively influence human life
- Analysis of the future possibilities of life on Mars
- Mars has the potential to support human life
- Evidence supporting the hypothesis of life on Mars
Feel free to reach out and learn more about thesis statements and relevant information to help you write a thesis. More so, we’ll write a variety of papers, from essays, homework, assignments, to dissertations, and guarantee quality essays with thesis statements that stand out.
Our website ordering process is straightforward, and we are available for any dissertation writing service you need. Talk to us and join thousands of satisfied clients who have worked with us. Do you need quality and reliable work? Feel free to talk to us any time, and we’ll sort you out.
Leave a Reply Cancel reply
Writing Life on Mars: Posthuman Imaginaries of Extraterrestrial Colonization and the NASA Mars Rover Missions
- First Online: 01 January 2022
Cite this chapter
- Jens Temmen 6
Part of the book series: Palgrave Studies in Life Writing ((PSLW))
527 Accesses
Focusing on three different life narratives and practices of Mars colonization—the NASA rover missions, the technoliberal campaign to settle the red planet by space entrepreneur Elon Musk, and the critical activist project “Planetary Personhood”—Jens Temmen analyzes how, in the context of climate change debates, human colonization of other planets has been reframed as inevitable and necessary to ensure the survival of humanity. Temmen’s contribution focuses on how these “astrofuturist” narratives negotiate the utopian vision of space colonization as a transformative posthuman experience of escape from the terrestrial limits placed on humanity, and it discusses whether life writing as a format allows for a thorough and critical posthuman inquiry of humanity’s anthropocentrism.
This is a preview of subscription content, log in via an institution to check access.
Access this chapter
Subscribe and save.
- Get 10 units per month
- Download Article/Chapter or eBook
- 1 Unit = 1 Article or 1 Chapter
- Cancel anytime
- Available as PDF
- Read on any device
- Instant download
- Own it forever
- Available as EPUB and PDF
- Compact, lightweight edition
- Dispatched in 3 to 5 business days
- Free shipping worldwide - see info
- Durable hardcover edition
Tax calculation will be finalised at checkout
Purchases are for personal use only
Institutional subscriptions
Similar content being viewed by others
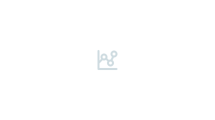
Scorched Earth: Discourses of Multiplanetarity, Climate Change, and Martian Terraforming in Finch and Once Upon a Time I Lived on Mars .
The Universe Decentered: Transcultural Perspectives on Astrobiology and Big History
Missions and Explorers: “Amundsen” as a Key to Reading Alice Munro’s Other Stories
Ganser, “Astrofuturism,” 36.
Ibid., 35, 37.
See Ganser, “Astrofuturism,” 36, 40. See Kilgore, “Astrofuturism,” 1, quoted in Ganser, “Astrofuturism,” 35.
Davenport, The Space Barons , 4, 123, 143–144.
See Ganser, “Astrofuturism,” 37, 40. See Redmond, “The Whiteness of Cinematic,” 348.
See Braidotti, The Posthuman , 9.
See Messeri, Placing Outer Space , 2.
See Vertesi, Seeing Like a Rover , 20.
Similar to the idea of “greenwashing” products as environmental-friendly, the concept of “double red washing” refers to how the privatization of the space industry is promoted by Musk and others as the only way to achieve the colonization of the red planet, and to how both the privatized space industry and Mars colonization are depicted as progressive measures aiming for greater social justice (see Marx, “Elon Musk is Planning”).
See Braidotti, The Posthuman , 43–44.
Opportunity’s sister rover “Spirit” had shut down in 2009 already.
See “‘My battery is low.’”
See Simon, “Opinion.”
See Butler, “Precariousness and Grievability.”
Butler analyzes how in times of war and crisis, the notion of a grievable death is a political issue of enormous significance. Focusing on 9/11 and the subsequent war in Iraq, Butler describes how public mourning can be used as a way of supporting the war effort and of constructing nationalist (non-)belonging, but also as a mode of dehumanization of the lives that are specifically constructed as ungrievable. Even though Butler’s work is deeply immersed in the specific context of warfare, her assumptions are valid for the way in which the rovers and their demise figure as a ledger for nationalistic sentiments of belonging and pride.
The playlist consists of the music that NASA staffers had sent to Opportunity along with its wake-up command after the storm had crippled him and includes songs such as David Bowie’s “Life on Mars?,” “Staying Alive” by the BeeGees, as well as Wham!‘s “Wake Me up Before You Go-Go.”
Simon, “Opinion.”
See Messeri, Placing Outer Space , 19.
Messeri, Placing Outer Space , 19.
See Messeri, Placing Outer Space , 5, 19, 34.
Braidotti, The Posthuman , 2.
Vertesi, Seeing Like a Rover , 20.
See Atanasoski and Vora, “Why the Sex Robot,” 2.
The way that the rovers seem to break barriers between technology, human, and animal, make them comparable to other posthuman icons, such as the cloned sheep Dolly, which, according to Braidotti, is an entity “no longer an animal but not yet fully a machine,” 74. The rovers, no longer a machine but not yet fully human, seem to be on that same spectrum.
Atanasoski and Vora, “Why the Sex Robot,” 6. See Markley, Dying Planet , 270.
Braidotti, The Posthuman , 43–44.
See Vertesi, Seeing Like a Rover , 7, 170–171.
Vertesi, Seeing Like a Rover , 25.
Ibid., 171.
Ibid., 176.
Keeling, “Queer OS,” 157.
See Braidotti, The Posthuman , 9, 124, 125–126. See Mbembe, Necropolitics .
Atanasoski and Vora, “Why the Sex Robot,” 1, 6. Atanasoski and Vora’s work focuses predominately on self-reproducing robots which are projected to be a vital part of the first steps to Martian colonization, but which have not yet been constructed or deployed (see ibid., 2). The way that NASA frames the current Mars rovers as links in human evolution, allows, I would argue, for a broadening of Atanasoski and Vora’s argument to apply here as well.
See Messeri, Placing Outer Space , 18, 68.
See Messeri, Placing Outer Space , 18. See Vertesi, Seeing Like a Rover , 31–32. A prominent and striking example is the influential essay by aerospace engineer Robert Zubrin, titled “The Significance of the Martian Frontier,” which advocates for Martian colonization through frontier discourses in general and by directly drawing on Frederick Jackson Turner’s infamous frontier thesis in particular, 13.
The concept of terra nullius is a core tenet of settler colonialism that relies on framing the Indigenous populations of newly “discovered” lands as less-than-human and therefore without claim to their own land (see Robertson, Conquest by Law ).
Atanasoski and Vora, “Why the Sex Robot,” 2.
See Temmen, “From HI-SEAS to Outer Space.”
See Saraf, “‘We’d Rather Eat Rocks.’”
See Messeri, Placing Outer Space , 68.
Atanasoski and Vora, “Why the Sex Robot,” 6.
Even though space agencies around the globe rely (and have relied for a long time) on private industry contractors, space travel is not a privatized business, but regulated on a national and international level. Private businesses can partner with national agencies, but they cannot conduct space travel on their own. While Musk and others have become important players as contractors, their vision includes completely privatizing space travel, which would, in their perspective, break a detrimental nation-state monopoly on space travel.
Davenport, The Space Barons , 4.
Ibid., 4–5.
See Wallace-Wells, The Uninhabitable Earth , 175–176.
Messeri, Placing Outer Space , 18.
Atanasoski and Vora, “Why the Sex Robot,” 11.
Davenport, The Space Barons , 1–2.
See Davenport, The Space Barons , 2.
Atanasoski and Vora, “Why the Sex Robot,” 1.
See Davenport, The Space Barons , 49–60, 117.
“When Slow Violence Sprints.”
Wallace-Wells, The Uninhabitable Earth , 176.
See Braidotti, The Posthuman , 5–6. See Chakrabarty, “The Climate of History,” 221–222.
See Heise, Sense of Planet , 25. See Atanasoski and Vora, “Why the Sex Robot,” 16.
Nonhuman Nonsense, “Planetary Personhood.”
See New Zealand Parliamentary Counsel Office, “Te Urewera Act 2014.”
See Atanasoski and Vora, “Why the Sex Robot,” 14.
Works Cited
Atanasoski, Neda, and Kalindi Vora. “Why the Sex Robot Becomes the Killer Robot – Reproduction, Care, and the Limits of Refusal.” Spheres: Journal for Digital Cultures 6 (2020): 1–16.
Google Scholar
Braidotti, Rosi. The Posthuman . Cambridge: polity, 2013.
Butler, Judith. “Precariousness and Grievability – When Is Life Grievable?” Accessed November 25, 2020. https://www.versobooks.com/blogs/2339-judith-butler-precariousness-and-grievability-when-is-life-grievable .
Chakrabarty, Dipesh. “The Climate of History: Four Theses.” Critical Inquiry 35 (2009): 197–222.
Article Google Scholar
Davenport, Christian. The Space Barons: Elon Musk, Jeff Bezos, and the Quest to Colonize the Cosmos . New York: Public Affairs, 2018.
Ganser, Alexandra. “Astrofuturism.” In Critical Terms in Future Studies, edited by Heike Paul, 35–43. London: Palgrave Macmillan, 2019.
Chapter Google Scholar
Heise, Ursula K. Sense of Place and Sense of Planet: The Environmental Imagination of the Global . Oxford: Oxford University Press, 2008.
Book Google Scholar
Keeling, Kara. “Queer OS.” Cinema Journal 53, no. 2 (2014): 152–157.
Markley, Robert. Dying Planet: Mars in Science and the Imagination . Durham: Duke University Press, 2005.
Marx, Paris. “Elon Musk is Planning for Climate Apocalypse.” Jacobin Magazine . Accessed December 1, 2020. https://jacobinmag.com/2020/01/elon-musk-climate-apocalypse-tesla-spacex .
Mbembe, Achille. Necropolitics . Durham: Duke University Press, 2019.
Messeri, Lisa. Placing Outer Space: An Earthly Ethnography of Other Worlds . Durham: Duke University Press, 2016.
“‘My battery low and it’s getting dark’: Mars Rover Opportunity’s Last Message to Scientists.” abc7chicago.com . Accessed September 21, 2020. https://abc7chicago.com/science/my-battery-is-low-and-its-getting-dark-opportunitys-last-message-to-scientists/5137455/ .
New Zealand Parliamentary Counsel Office. “Te Urewera Act 2014.” Accessed January 26, 2021. https://www.legislation.govt.nz/act/public/2014/0051/latest/DLM6183601.html .
Nonhuman Nonsense. “Planetary Personhood.” Accessed November 27, 2020. https://planetarypersonhood.com .
Redfield, Peter. Space in the Tropics: From Convicts to Rockets in French Guiana . Berkeley: University of California Press, 2000.
Redmond, Sean. “The Whiteness of Cinematic Outer Space.” In The Palgrave Handbook of Society, Culture and Outer Space , edited by Peter Dickens and James S. Ormrod, 337–354. London: Palgrave Macmillan, 2016.
Robertson, Lindsay G. Conquest by Law: How the Discovery of America Dispossessed Indigenous Peoples of Their Lands . Oxford: Oxford University Press, 2005.
Saraf, Aanchal. “‘We’d Rather Eat Rocks’: Contesting the Thirty-Meter Telescope in a Struggle Over Science and Sovereignty in Hawaiʻi.” In “American Territorialities,” edited by Jens Temmen and Nicole Waller. Special Forum, Journal for Transnational American Studies 11, vol. 1 (2020): 151–175.
Sheller, Mimi. “Space Age Tropics.” In Surveying the American Tropics: A Literary Geography From New York to Rio , edited by Maria Cristina Fumagalli, Peter Hulme, Owen Robinson, and Lesley Wylie, 131–158. Liverpool: Liverpool University Press, 2017.
Simon, Scott. “Opinion: Good Night, Oppy, A Farewell to NASA’s Mars Rover.” Accessed September 21, 2020. https://www.npr.org/2019/02/16/695293679/opinion-good-night-oppy-a-farewell-to-nasas-mars-rover .
Temmen, Jens. “From HI-SEAS to Outer Space: Discourses of Water and Territory in U.S. Pacific Imperialism and Representations of U.S. Mars Colonization.” In Maritime Mobilities in Anglophone Literature and Culture , edited by Alexandra Ganser and Charne Lavery. Palgrave Macmillan. Forthcoming 2022.
Vertesi, Janet. Seeing Like A Rover: How Robots, Teams, and Images Craft Knowledge of Mars . Chicago: University of Chicago Press, 2015.
Wallace-Wells, David. The Uninhabitable Earth: Life After Warming . New York: Tim Duggan Books, 2019.
“When Slow Violence Sprints: Interview with Rob Nixon.” Harvard University Press Blog . Accessed November 24, 2020. https://harvardpress.typepad.com/hup_publicity/2013/11/when-slow-violence-sprints-rob-nixon.html .
Zubrin, Robert. “The Significance of the Martian Frontier.” In Strategies for Mars: A Guide to Human Exploration , edited by Carol R. Stoker and Carter Emmart, 13–26. San Diego: American Astronautical Society, 1996.
Download references
Author information
Authors and affiliations.
American Studies Department, Heinrich-Heine-University, Düsseldorf, Germany
Jens Temmen
You can also search for this author in PubMed Google Scholar
Corresponding author
Correspondence to Jens Temmen .
Editor information
Editors and affiliations.
American Studies, Faculty of Philology & History, University of Augsburg, Augsburg, Germany
American Studies, Faculty of Humanities & Cultural Studies, University of Wuppertal, Wuppertal, Germany
Lea Espinoza Garrido
Linda M. Hess
Rights and permissions
Reprints and permissions
Copyright information
© 2021 The Author(s), under exclusive license to Springer Nature Switzerland AG
About this chapter
Temmen, J. (2021). Writing Life on Mars: Posthuman Imaginaries of Extraterrestrial Colonization and the NASA Mars Rover Missions. In: Batzke, I., Espinoza Garrido, L., Hess, L.M. (eds) Life Writing in the Posthuman Anthropocene. Palgrave Studies in Life Writing. Palgrave Macmillan, Cham. https://doi.org/10.1007/978-3-030-77973-3_8
Download citation
DOI : https://doi.org/10.1007/978-3-030-77973-3_8
Published : 01 January 2022
Publisher Name : Palgrave Macmillan, Cham
Print ISBN : 978-3-030-77972-6
Online ISBN : 978-3-030-77973-3
eBook Packages : Literature, Cultural and Media Studies Literature, Cultural and Media Studies (R0)
Share this chapter
Anyone you share the following link with will be able to read this content:
Sorry, a shareable link is not currently available for this article.
Provided by the Springer Nature SharedIt content-sharing initiative
- Publish with us
Policies and ethics
- Find a journal
- Track your research
Thank you for visiting nature.com. You are using a browser version with limited support for CSS. To obtain the best experience, we recommend you use a more up to date browser (or turn off compatibility mode in Internet Explorer). In the meantime, to ensure continued support, we are displaying the site without styles and JavaScript.
- View all journals
- Explore content
- About the journal
- Publish with us
- Sign up for alerts
- Open access
- Published: 21 February 2023
Life on Mars, can we detect it?
- Carol R. Stoker ORCID: orcid.org/0000-0001-7265-292X 1
Nature Communications volume 14 , Article number: 807 ( 2023 ) Cite this article
5661 Accesses
2 Citations
310 Altmetric
Metrics details
- Astrobiology
- Biochemistry
Searching for evidence of life on Mars is a major impetus for exploration. A new study published in Nature Communications finds that current Mars mission instruments lack the essential sensitivity to identify life traces in Chilean desert samples that strongly resemble the martian area currently under study by NASA’s Perseverance rover.
Almost half a century ago the NASA Viking landers searched for evidence of life in Mars soils by attempting to detect active metabolism and measuring organic compounds by heating to vaporize them for detection via Gas Chromatography Mass Spectrometry (GC-MS). When no organic compounds were detected even at levels of parts per billion 1 , this provided a puzzle. Since organic compounds are continuously delivered to Mars by meteorites, comets, and interplanetary dust particles they should be ubiquitous and comprise up to 2% of Mars soil 2 . But where are they? This curious lack of organics argued both against a biological interpretation for results of the metabolism experiments, and for the presence of strong soil oxidants that actively destroy these compounds 3 . However, decades later the Viking GC-MS results were explained by the presence of high levels of perchlorate salts in the Martian soil 4 that oxidized organics during pyrolysis in the Viking GC-MS instrument 5 .
While Viking lander results seemed unpromising for surface life in the cold, dry martian conditions, data from Viking orbiters and later missions showed that Mars had surface liquid water early in its history (3 to 4 billion years ago) 6 where life may have thrived. This led later NASA rover missions to seek evidence of ancient habitable environments hosting organic remnants of life. The SAM ( Sample Analysis on Mars ) instrument on the Curiosity rover detected organics in ancient lakebed deposits 7 , 8 , but they are found at low levels (parts per billion) and appear to be highly altered. The Perseverance rover, now exploring an ancient illuvial fan river delta, has detected aromatic organic compounds using fluorescence spectroscopy 9 . But in these cases, the simple organics detected could have been delivered to Mars from space so proving a biological origin is impossible.
The paper by Azua-Bustos et al. 10 in Nature Communications describes analysis of samples collected from “Red Stone”, an alluvial fan river delta more than 100 million years old in the Atacama Desert of Chile, Earth’s oldest and driest desert. Red Stone has similar geology to the delta area currently being studied by NASA’s Perseverance rover 11 (Fig. 1 ). The Red Stone location (near the ocean) frequently experiences fogs that provide water for sparse but active microbial life that was detected by DNA extraction and gene sequencing, microscopy, and growth of a few microbial strains cultured from the samples. Most microbes identified consist of “microbial dark matter” i.e., genetic information is from organisms that have not yet been described.
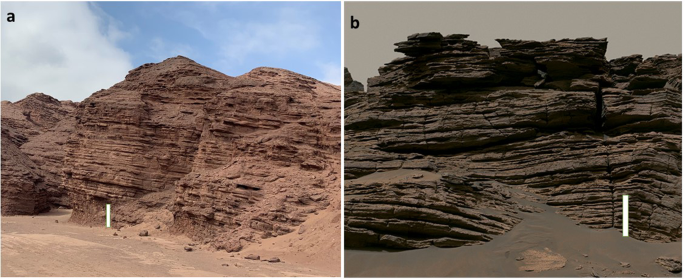
a Red Stone deposit from panorama image courtesy of Armando Azua-Bustos. b A section of the panoramic composite image [image PIA24921_MAIN-20k.jpg] of the Jezero delta captured by the MastCam-Z camera on the Perseverance Rover in June 2022 15 (Courtesy NASA/JPL-Caltech/ASU/MSSS). The height of both white scale bars are ~2 m.
Samples from Red Stone were also analyzed by instruments that simulate those used on Mars, but even finding organics in these samples was challenging. A GC-MS instrument comparable to but ten times more sensitive than Curiosity rover’s SAM instrument was barely able to detect specific biogenic organic compounds at the limit of detection, suggesting that SAM could not have detected them. There was more success with organic detection after sample extraction with a powerful solvent that was also implemented on the SAM instrument. Indeed, the SAM instrument detected organics in both sedimentary mudstones and dune sands on Mars by using solvent extraction and derivatization (a chemical treatment to make organic compounds easier to detect) 8 .
Red Stone samples were analyzed with a testbed of the MOMA (Mars Organic Molecular Analysis) instrument 12 planned for the European ExoMars rover payload. No organics were detected when flash pyrolysis was used, but a few organic molecules were identified in evaporite samples when they were first extracted with a solvent and derivatized.
Red Stone samples were also analyzed with the SOLID-LDChip (Signs of Life Detector-Life Detector Chip) 13 , an instrument based on the technology of immunoassay that is commonly used in biomedicine. SOLID was designed for life detection on Mars but no scheduled missions plan to use it. Interestingly, the LDChip detected evidence of cyanobacteria that were not seen in the modern microbiota so Azua-Bustos et al. 10 posit these biosignatures were deposited along with the delta sediments at least 100 million years ago.
This Red Stone sample analysis 10 shows how critical it is to test instruments designed for life detection on other planets by using samples from relevant Earth analogs prior to selecting them for flight missions. If the biosignatures can’t be detected in Earth samples, where both current and ancient life is clearly documented, we should not expect these instruments to be capable of detecting evidence of life from Mars’ early history.
Azua-Bustos et al. 10 argue that detection of ancient life signatures will require sample analysis in sophisticated terrestrial laboratories. This same view has led to the current plans for searching for life: the Perseverance rover is collecting samples that are to be retrieved and brought to Earth by a future mission 14 . But any biological activity in these samples presumably took place billions of years ago, and only a few small samples can be brought to Earth for study. It remains to be seen if unambiguous signatures of life can be found in those limited samples. We must be cautious about interpreting absence of strong evidence of life as evidence of its absence!
Biemann, K. & Lavoi, J. M. Jr Some final conclusions and supporting experiments related to the search for organic compounds on the surface of Mars. J. Geophys. Res. 84 , 8385–8390 (1979).
Article CAS ADS Google Scholar
Flynn, G. J. & McKay, D. S. An assessment of the meteoritic contribution to the Martian soil. J. Geophys. Res. 95 , 14,497–14,509 (1990).
Article ADS Google Scholar
Klein, H. P. The Viking biological experiments on Mars. Icarus 34 , 666–674 (1978).
Hecht, M. H. et al. Detection of perchlorate and the soluble chemistry of martian soil: findings from the Phoenix Mars Lander. Science 325 , 64–67 (2009).
Article CAS ADS PubMed Google Scholar
Navarro‐González, R. et al. Reanalysis of the Viking results suggests perchlorate and organics at midlatitudes on Mars. J. Geophys. Res. 115 , E12010 (2010).
Carr, M. H. & Head, J. W. III Geologic history of Mars. Earth Planet. Sci. Lett. 294 , 185–203 (2010).
Eigenbrode, J. L. et al. Organic matter preserved in 3-billion-year-old mudstones at Gale crater, Mars. Science 360 , 1096–1101 (2018).
Millan, M. et al. Sedimentary organics in Glen Torridon, Gale Crater, Mars: Results from the SAM instrument suite and supporting laboratory analyses. J. Geophys. Res. Planets 127 , e2021JE007107 (2022).
Scheller, E. L. et al. Aqueous alteration processes in Jezero Crater Mars- implications for organic geochemistry. Science 378 , 1105–1110 (2022).
Azua-Bustos, A. et al. Dark microbiome and extremely low organics in an Atacama fossil river delta unveil the limits of life detection on Mars. Nat. Commun. this issue . https://doi.org/10.1038/s41467-023-36172-1 (2023).
Farley, K. A. et al. Aqueously altered igneous rocks sampled on the floor of Jezero Crater. Science 377 , 1512 (2022).
Article Google Scholar
Goesmann, F. et al. The Mars Organic Molecule Analyzer (MOMA) instrument: characterization of organic material in martian sediments. Astrobiology 17 , 655–685 (2017).
Article CAS ADS PubMed PubMed Central Google Scholar
Parro, V. et al. SOLID3, a multiplex antibody microarray-based optical sensor instrument for in situ life detection in planetary exploration. Astrobiology 11 , 15–28 (2011).
Haltigen, T. et al. Rationale and Proposed Design for a Mars Sample Return (MSR) Science Program. Astrobiology 22 , S27–S55 (2022).
A small segment of a panorama comprised of Perseverance Rover MastCam-Z images (image PIA24921_Main-20k.jpg) taken June 2022. The full panoramic image is available for download at https://mars.nasa.gov/resources/26978/detailed-panorama-of-mars-jezero-crater-delta/ .
Download references
Author information
Authors and affiliations.
NASA Ames Research Center, Space Science Division, MS 245-3, Moffett Field, California, CA, 94035, USA
Carol R. Stoker
You can also search for this author in PubMed Google Scholar
Contributions
CRS is the sole author.
Corresponding author
Correspondence to Carol R. Stoker .
Ethics declarations
Competing interests.
The author declares no competing interests.
Additional information
Publisher’s note Springer Nature remains neutral with regard to jurisdictional claims in published maps and institutional affiliations.
Rights and permissions
Open Access This article is licensed under a Creative Commons Attribution 4.0 International License, which permits use, sharing, adaptation, distribution and reproduction in any medium or format, as long as you give appropriate credit to the original author(s) and the source, provide a link to the Creative Commons license, and indicate if changes were made. The images or other third party material in this article are included in the article’s Creative Commons license, unless indicated otherwise in a credit line to the material. If material is not included in the article’s Creative Commons license and your intended use is not permitted by statutory regulation or exceeds the permitted use, you will need to obtain permission directly from the copyright holder. To view a copy of this license, visit http://creativecommons.org/licenses/by/4.0/ .
Reprints and permissions
About this article
Cite this article.
Stoker, C.R. Life on Mars, can we detect it?. Nat Commun 14 , 807 (2023). https://doi.org/10.1038/s41467-023-36176-x
Download citation
Received : 14 December 2022
Accepted : 09 January 2023
Published : 21 February 2023
DOI : https://doi.org/10.1038/s41467-023-36176-x
Share this article
Anyone you share the following link with will be able to read this content:
Sorry, a shareable link is not currently available for this article.
Provided by the Springer Nature SharedIt content-sharing initiative
Quick links
- Explore articles by subject
- Guide to authors
- Editorial policies
Sign up for the Nature Briefing newsletter — what matters in science, free to your inbox daily.

“Is there life on Mars?” is a question people have asked for more than a century. But in order to finally get the answer, we have to know what to look for and where to go on the planet to look for evidence of past life. With the Perseverance rover set to land on Mars on February 18, 2021, we are finally in a position to know where to go, what to look for, and knowing whether there is, or ever was, life on the Red Planet.
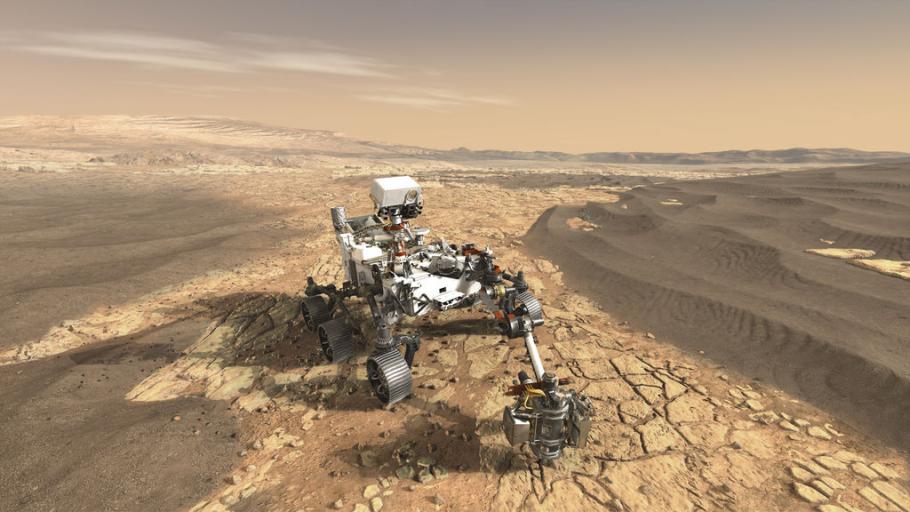
Science fiction aside, we know that there were not ancient civilizations or a population of little green people on Mars. So, what sort of things do we need to look for to know whether there was ever life on Mars? Fortunately, a robust Mars exploration program, including orbiters, landers, and rovers, has enabled detailed mapping of the planet and constrained important information about the environment.
We now know that there were times in the ancient past on Mars when conditions were wetter and at least a little warmer than the fairly inhospitable conditions that are present today. And there were once habitable environments that existed on the surface. For example, the Curiosity rover has shown that more than three billion years ago, Gale crater was the location of a lake that held water likely suitable for sustaining life. Armed with information about the conditions and chemical environments on the surface, the Perseverance rover is outfitted with a science payload of instruments finely tuned for extracting information related to any biosignatures that might be present and signal the occurrence of life .
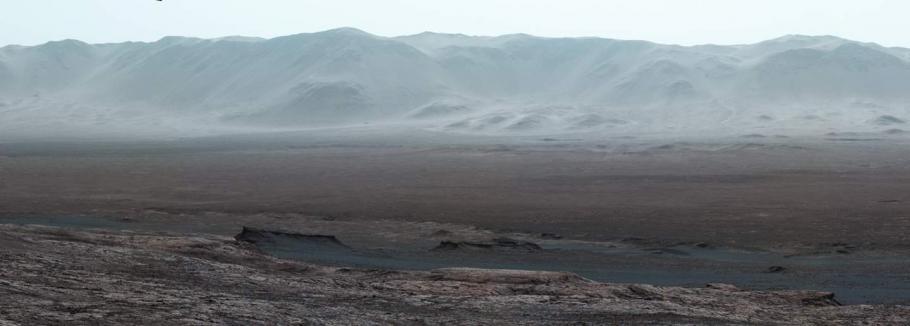
Panoramic view of the interior and rim of Gale crater. Image generated from pictures captured by the Curiosity rover.
But where should we go on Mars to maximize the chances of accessing the rocks most likely to have held and preserve any evidence of past life? To get at that answer, I co-led a series of workshops attended by the Mars science community to consider various candidate landing sites and help determine which one had the highest potential for preserving evidence of past life. Using data from Mars orbiters coupled with more detailed information from landers and rovers, we started with around thirty candidate sites and narrowed the list over the course of four workshops and five years. Some sites were clearly less viable than others and were weeded out fairly quickly. But once the discussion focused on a couple of different types of potentially viable sites, the process became much tougher. In the end, the science community felt—and the Perseverance mission and NASA agreed—that Jezero crater was the best place to look for evidence of past life on Mars.
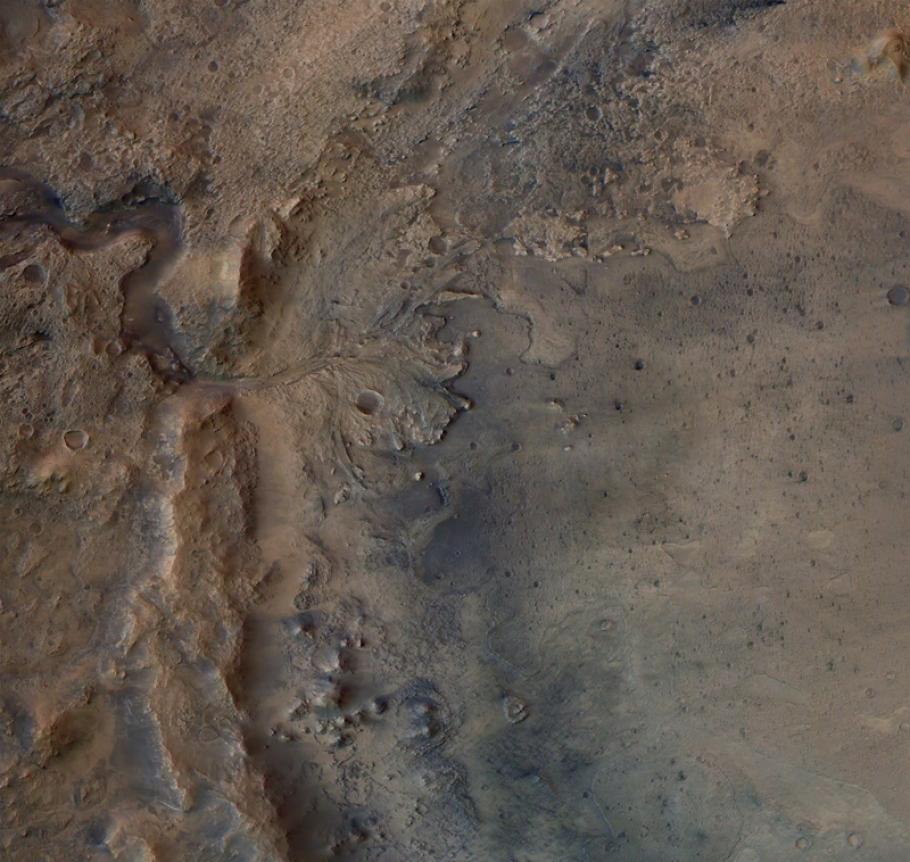
This image shows the remains of an ancient delta in Mars' Jezero Crater, which NASA's Perseverance Mars rover will explore for signs of fossilized microbial life. The image was taken by the High Resolution Stereo Camera aboard the ESA (European Space Agency) Mars Express orbiter. The European Space Operations Centre in Darmstadt, Germany, operates the ESA mission. The High Resolution Stereo Camera was developed by a group with leadership at the Freie Universitat Berlin.
What is so special about Jezero crater and where is it? Jezero crater is ~30 miles (~49 km) across, was formed by the impact of a large meteorite, and is located in the northern hemisphere of Mars (18.38°N 77.58°E) on the western margin of the ancient and much larger Isidis impact basin. But what makes it special relates to events that happened 3.5 billion years ago when water was more active on the surface of Mars than it is today. Ancient rivers on the western side of Jezero breached the crater rim and drained into the crater, forming a river delta and filling the crater with a lake. From the study of river deltas on the Earth, we know that they typically build outwards into lakes as sediment carried by the associated river enters the lake, slows down, and is deposited. As this process continues, the delta builds out over the top of lake beds and can bury and preserve delicate and subtle signatures of past life. These “biosignatures” are what Perseverance will be looking for when it lands on the floor of the crater and explores the ancient lake beds and nearby delta deposits.
Perseverance will use its instruments to look for signs of ancient life in the delta and lake deposits in Jezero crater and will hopefully allow us to finally answer the question of whether there was ever life on Mars. In addition, Perseverance will begin the process of collecting samples that could one day be returned to Earth. The importance of sample return cannot be overstated. Whether or not evidence of past life is found by Perseverance’s instruments, the legacy enabled by samples the rover collects will be the “scientific gift that keeps on giving”. Once returned to Earth by a future mission, these Mars samples can be subjected to more detailed analysis by a much wider set of instruments than can be carried by Perseverance . Moreover, sample archiving can preserve material for future analysis here on Earth by new and/or more detailed instruments that may not yet exist. So even if Perseverance does not find evidence of past life, it will collect samples that, once returned to Earth, could provide new insight into the evolution of Mars and whether there was ever life on the Red Planet.
We rely on the generous support of donors, sponsors, members, and other benefactors to share the history and impact of aviation and spaceflight, educate the public, and inspire future generations. With your help, we can continue to preserve and safeguard the world’s most comprehensive collection of artifacts representing the great achievements of flight and space exploration.
- Get Involved
- Host an Event
Thank you. You have successfully signed up for our newsletter.
Error message, sorry, there was a problem. please ensure your details are valid and try again..
- Free Timed-Entry Passes Required
- Terms of Use
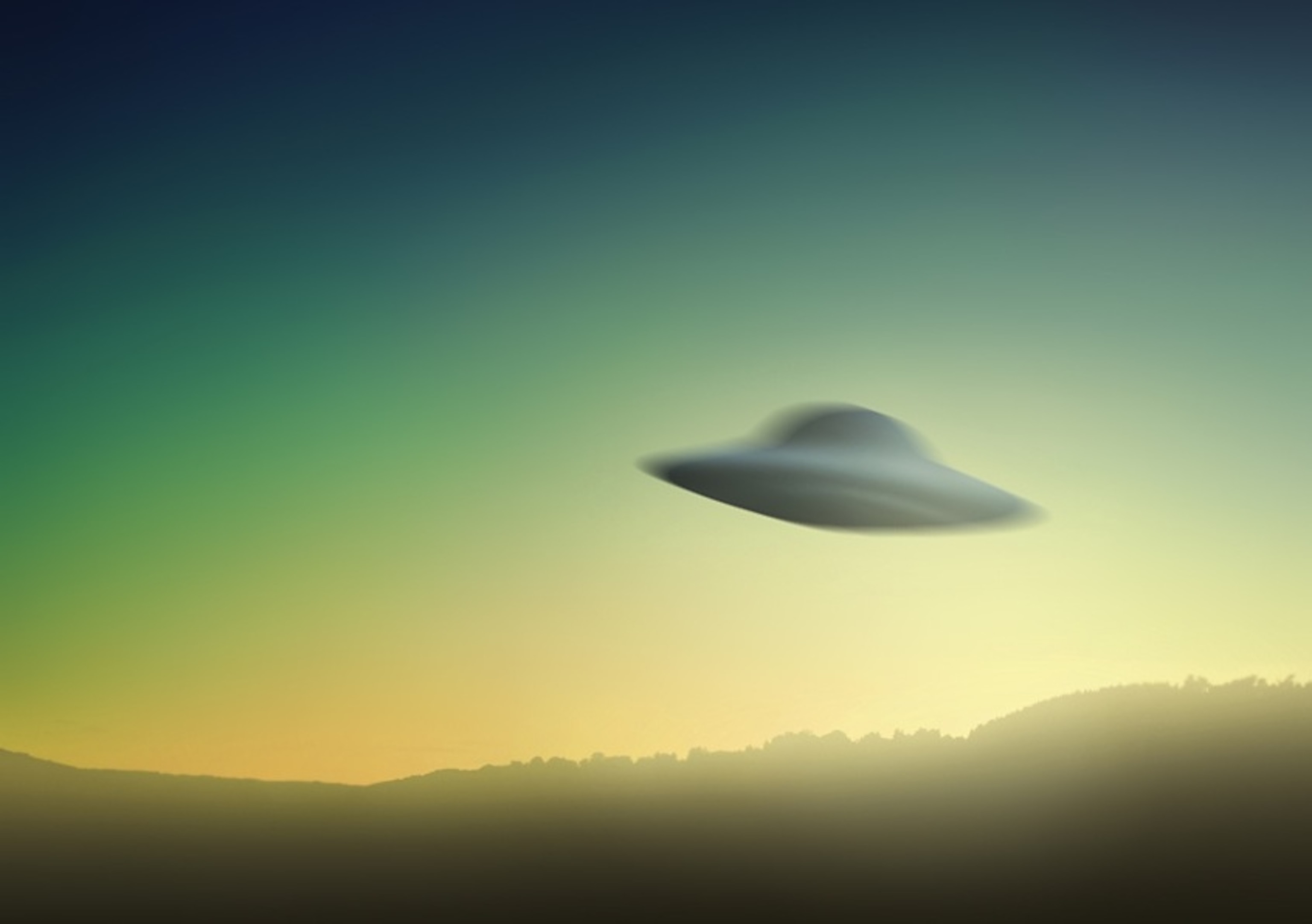
Life on Mars and the Imagination of Scientists
Around this time seven years ago I was trying to figure out a topic for my Master’s thesis. It could have been anything at all, so long as it fit under the wide umbrella of science writing. After a few dead ends (sumo wrestling, Amish science) I finally chose to write about the hunt for life on Mars.
My advisor wasn’t keen on the idea, and it was way, way out of my wheelhouse. But I pushed on anyway, for three reasons I can remember. Astrobiology has only been considered a legitimate scientific endeavor since the ’60s. So every study felt fresh and exciting. It’s also inherently multidisciplinary — requiring geologists, climate scientists, astrophysicists, engineers, DNA experts, microbiologists and even philosophers — which meant my story would have lots of different voices. Perhaps most important, all of those voices are focused on one Big Question: Are we alone in the universe?
For the same reasons, astrobiology is perfect for science education, or so argues a new study in the journal Astrobiology . Researchers from the University of New South Wales, in Sydney, Australia, surveyed high schoolers before and after completing a one-day museum program in which they pretended to be scientists involved in Mars rover missions . The study found that this simulation corrected some of the students’ misperceptions about science and scientists.
These effects, though, were small. Overall the study was quite depressing, especially on one point: Even after completing the program, around two-thirds of the students said they didn’t think that scientists are creative or use their imaginations.
This is a massive problem. And fixing it will take a lot more than flashy rovers and the promise of aliens.
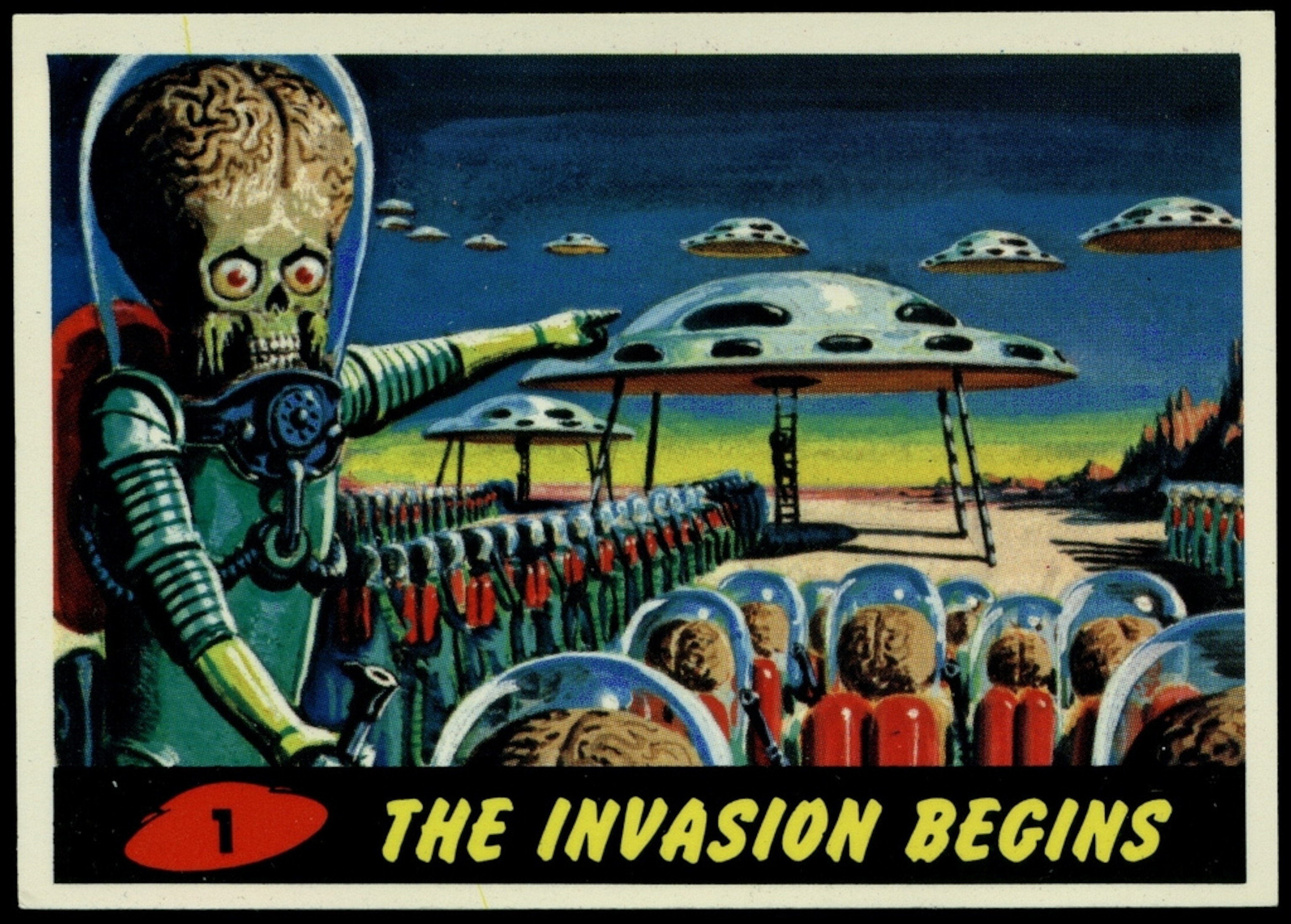
Somebody in the humanities might legitimately ask why I think this is a problem. Some kids like science, some like art, big deal?
Liking science isn’t the issue. Consider a 2007 survey of 15-year-olds from some 25 countries. The students rated how much they agreed with a bunch of statements about science (from 1, Disagree, to 4, Agree). In every country surveyed, the average rating of “School science is interesting” was above the neutral score of 2.5. In the new Australian study, too, the teenagers liked science. They were recruited to the study, in fact, because they had signed up for a one-day museum program.
But kids can’t picture themselves doing science for a living. Another statement in that same 2007 survey was, “I would like to become a scientist.” The average rating from developed countries* was around 2 for boys and a dismal 1.5 for girls. Similarly, a 2010 study followed 33 students in California who in 10th grade said they were “very interested” in a science career. By 12th grade, 45 percent had lost interest.
So how come kids who like science don’t want to become scientists? One big reason, according to many studies, is they don’t think it’s a creative job. In-depth interviews with high school and college students (whether from New York , Europe , or New Zealand ) have revealed that the students often believe that science and creativity are incompatible. Seriously.
This couldn’t be farther from the truth, of course, and that’s the lesson the Australian researchers were hoping to get across with the Mars programs. The researchers surveyed 230 teenagers who participated in one of two museum programs: Pathways to Space at the Powerhouse Museum, in Sydney, and Mission to Mars at the Victorian Space Science Education Centre, in Melbourne. Both programs seem super-fun. In Pathways, students plan a rover mission and then execute it in a “Mars Yard” that looks strikingly like the real thing (which you can see in this short video ). In Mission to Mars, students role-play being astronauts or mission control engineers, and analyze data picked up by a rover in a simulated martian crater.
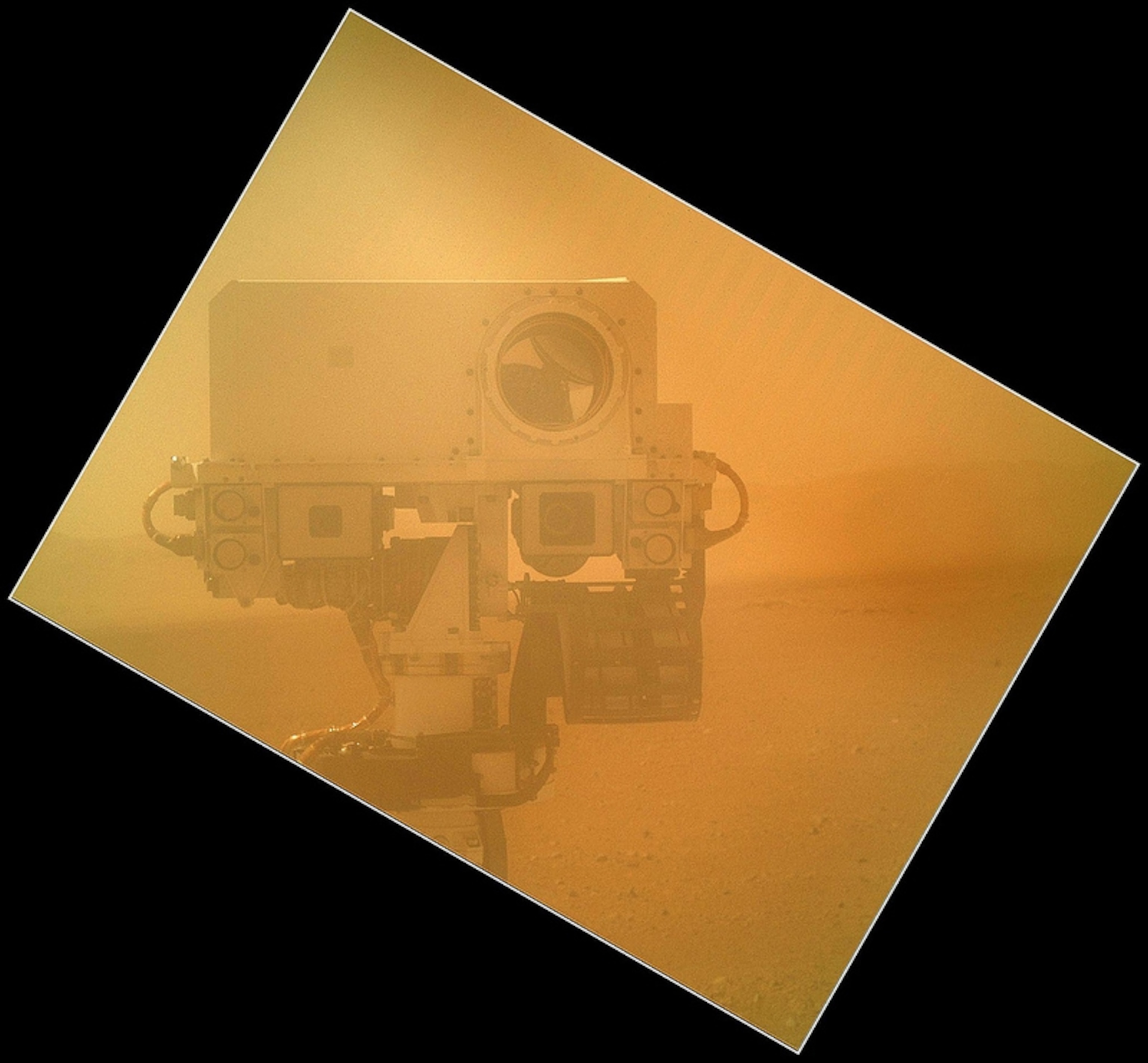
The students’ responses perfectly illustrate the broader issues in science education that I outlined above. Before the one-day program, only 17 percent of the kids agreed or strongly agreed with the statement, “Science is boring.” Yet just 15 percent agreed or strongly agreed with the statement, “I want to become a scientist,” and only about 30 percent agreed or strongly agreed that scientists use their imagination and creativity throughout their investigations. After the day with the rovers, there was a statistically significant increase in the creativity rating, to about 35 percent. But there was no change in the number of kids who wanted to be scientists.
My intention here isn’t to disparage what seems like a wonderful outreach program — if I lived in Australia I’d try to sign up for it myself. It would be crazy to expect a day-long field trip, however awesome, to immediately change a teenager’s career plans or philosophical outlook.
Still, the reason the program is great isn’t because it introduces kids to the exciting, multidisciplinary and provocative field of astrobiology. All fields of science, after all, are similarly wondrous and inspirational.
What we need to focus on instead is why the imagination of scientists doesn’t get through to most of the world’s young minds, even to those who are interested in science .
Are science journalists not describing it? Are science teachers ignoring it? Are scientists themselves too shy or modest or data-centric to spend much time talking about their creative process?
How can we fix this?
Interestingly, ratings from students in developing countries were much higher, around 3.
Images from Ben Heine , cigcardpix , and NASA Goddard
- Best of the World
- Environment
- Paid Content
History & Culture
- History & Culture
- Terms of Use
- Privacy Policy
- Your US State Privacy Rights
- Children's Online Privacy Policy
- Interest-Based Ads
- About Nielsen Measurement
- Do Not Sell or Share My Personal Information
- Nat Geo Home
- Attend a Live Event
- Book a Trip
- Inspire Your Kids
- Shop Nat Geo
- Visit the D.C. Museum
- Learn About Our Impact
- Support Our Mission
- Advertise With Us
- Customer Service
- Renew Subscription
- Manage Your Subscription
- Work at Nat Geo
- Sign Up for Our Newsletters
- Contribute to Protect the Planet
Copyright © 1996-2015 National Geographic Society Copyright © 2015-2024 National Geographic Partners, LLC. All rights reserved
NTRS - NASA Technical Reports Server
Available downloads, related records.

Assessment of Mars Science and Mission Priorities (2003)
Chapter: 13. conclusions, 13 conclusions.
It is humankind’s nature to explore our surroundings if it can be done. Fifty years ago, exploring Mars was not one of the things anyone could do. Those who were curious had to be content with fuzzy images of the planet, quivering in the oculars of telescopes. But that is far from the case today. Forty years ago, spacecraft began to be sent to the planets, and since then, the art of space exploration has become increasingly refined and discoveries have multiplied. We now have the capability, in principle, of reaching and exploring any object in the solar system. At the top of the list of targets of exploration is Mars, the most Earth-like, most accessible, most hospitable, and most intriguing of the planets. Two years ago, in October 2000, NASA recognized this by setting the study of Mars apart in a structured Mars Exploration Program. The present document reports on COMPLEX’s study of the program.
COMPLEX has compared the elements of the Mars Exploration Program with the research objectives for Mars that have been stressed by advisory panels, including this one, for more than 23 years. The committee found that correspondence between the two is not perfect. Currently, NASA focuses on the search for life, and its prerequisite, water, as the main drivers for Mars research, and has favored missions and experiments that support these goals. The space agency is not now in a position to ask direct questions about life on Mars, and has not been since the Viking mission in the 1970s, but the missions supported are designed to find the areas most promising for water and life, and to investigate in situ their chemical and petrographic potential for extant or fossil life.
Since NASA operates within budget constraints, this emphasis on one particular scientific objective necessarily comes at the expense of others. COMPLEX considered the question of whether NASA’s priorities are too heavily skewed toward life-related investigations. The committee decided, however, that this is not the case. The emphasis on life is well justified; the life-related investigations that are planned range over so much of Mars science that they will result in broad and comprehensive gains in our knowledge; and the areas most neglected as a consequence of this emphasis (see Chapter 12 ) will, to some extent, be investigated by projected missions of our international partners.
COMPLEX endorses the program NASA has set up, though the committee has also pointed out several areas of high scientific priority that the program does not address. This report stresses the uniquely important role of sample return in a program of Mars research, and urges that sample-return missions be performed as early as possible. Discussions and recommendations related to sample return appear in Chapters 7 and 12 . A more general review of the conclusions of this report is contained in the Executive Summary.

FIGURE 13.1 The study of Mars has come very far. This map is a reminder of how the planet was perceived in 1967. SOURCE: Mariner 69 Mars Chart, NASA MEC-2.
Our understanding of the most Earth-like planet beyond our own has increased dramatically in 35 years of spacecraft research (see Figure 13.1). Most of us will live to see an even greater increment of knowledge result from execution of the Mars Exploration Program that this report describes.
Within the Office of Space Science of the National Aeronautics and Space Administration (NASA) special importance is attached to exploration of the planet Mars, because it is the most like Earth of the planets in the solar system and the place where the first detection of extraterrestrial life seems most likely to be made. The failures in 1999 of two NASA missions—Mars Climate Orbiter and Mars Polar Lander—caused the space agency's program of Mars exploration to be systematically rethought, both technologically and scientifically. A new Mars Exploration Program plan (summarized in Appendix A) was announced in October 2000. The Committee on Planetary and Lunar Exploration (COMPLEX), a standing committee of the Space Studies Board of the National Research Council, was asked to examine the scientific content of this new program. This goals of this report are the following:
-Review the state of knowledge of the planet Mars, with special emphasis on findings of the most recent Mars missions and related research activities;
-Review the most important Mars research opportunities in the immediate future;
-Review scientific priorities for the exploration of Mars identified by COMPLEX (and other scientific advisory groups) and their motivation, and consider the degree to which recent discoveries suggest a reordering of priorities; and
-Assess the congruence between NASA's evolving Mars Exploration Program plan and these recommended priorities, and suggest any adjustments that might be warranted.
READ FREE ONLINE
Welcome to OpenBook!
You're looking at OpenBook, NAP.edu's online reading room since 1999. Based on feedback from you, our users, we've made some improvements that make it easier than ever to read thousands of publications on our website.
Do you want to take a quick tour of the OpenBook's features?
Show this book's table of contents , where you can jump to any chapter by name.
...or use these buttons to go back to the previous chapter or skip to the next one.
Jump up to the previous page or down to the next one. Also, you can type in a page number and press Enter to go directly to that page in the book.
Switch between the Original Pages , where you can read the report as it appeared in print, and Text Pages for the web version, where you can highlight and search the text.
To search the entire text of this book, type in your search term here and press Enter .
Share a link to this book page on your preferred social network or via email.
View our suggested citation for this chapter.
Ready to take your reading offline? Click here to buy this book in print or download it as a free PDF, if available.
Get Email Updates
Do you enjoy reading reports from the Academies online for free ? Sign up for email notifications and we'll let you know about new publications in your areas of interest when they're released.
- Ask An Astrobiologist
- Resources Graphic Histories Coloring Pages Heroes Posters Life in the extremes Digital Backgrounds SciComm Guild

Life on Mars: A Definite Possibility
Was Mars once a living world? Does life continue, even today, in a holding pattern, waiting until the next global warming event comes along? Many people would like to believe so. Scientists are no exception. But so far no evidence has been found that convinces even a sizable minority of the scientific community that the red planet was ever home to life. What the evidence does indicate, though, is that Mars was once a habitable world . Life, as we know it, could have taken hold there.
The discoveries made by NASA ’s Opportunity rover at Eagle Crater earlier this year (and being extended now at Endurance Crater) leave no doubt that the area was once ‘drenched’ in water . It might have been shallow water. It might not have stuck around for long. And billions of years might have passed since it dried up. But liquid water was there, at the martian surface, and that means that living organisms might have been there, too.
So suppose that Eagle Crater – or rather, whatever land formation existed in its location when water was still around – was once alive. What type of organism might have been happy living there?
Probably something like bacteria. Even if life did gain a foothold on Mars, it’s unlikely that it ever evolved beyond the martian equivalent of terrestrial single-celled bacteria. No dinosaurs; no redwoods; no mosquitoes – not even sponges, or tiny worms. But that’s not much of a limitation, really. It took life on Earth billions of years to evolve beyond single-celled organisms. And bacteria are a hardy lot. They are amazingly diverse, various species occupying extreme niches of temperature from sub-freezing to above-boiling; floating about in sulfuric acid; getting along fine with or without oxygen. In fact, there are few habitats on Earth where one or another species of bacterium can’t survive.
What kind of microbe, then, would have been well adapted to the conditions that existed when Eagle Crater was soggy? Benton Clark III , a Mars Exploration Rover ( MER ) science team member, says his “general favorite” candidates are the sulfate-reducing bacteria of the genus Desulfovibrio . Microbiologists have identified more than 40 distinct species of this bacterium.
Eating Rocks
We tend to think of photosynthesis as the engine of life on Earth. After all, we see green plants nearly everywhere we look and virtually the entire animal kingdom is dependent on photosynthetic organisms as a source of food. Not only plants, but many microbes as well, are capable of carrying out photosynthesis. They’re photoautotrophs: they make their own food by capturing energy directly from sunlight.
But Desulfovibrio is not a photoautotroph; it’s a chemoautotroph. Chemoautotrophs also make their own food, but they don’t use photosynthesis to do it. In fact, photosynthesis came relatively late in the game of life on Earth. Early life had to get its energy from chemical interactions between rocks and dirt, water, and gases in the atmosphere. If life ever emerged on Mars, it might never have evolved beyond this primitive stage.
Desulfovibrio makes its home in a variety of habitats. Many species live in soggy soils, such as marshes and swamps. One species was discovered all snug and cozy in the intestines of a termite. All of these habitats have two things in common: there’s no oxygen present; and there’s plenty of sulfate available.
Sulfate reducers, like all chemoautotrophs, get their energy by inducing chemical reactions that transfer electrons between one molecule and another. In the case of Desulfovibrio, hydrogen donates electrons, which are accepted by sulfate compounds. Desulfovibrio, says Clark, uses “the energy that it gets by combining the hydrogen with the sulfate to make the organic compounds” it needs to grow and to reproduce.
The bedrock outcrop in Eagle Crater is chock full of sulfate salts. But finding a suitable electron donor for all that sulfate is a bit more troublesome. “My calculations indicate [that the amount of hydrogen available is] probably too low to utilize it under present conditions,” says Clark. “But if you had a little bit wetter Mars, then there [would] be more water in the atmosphere, and the hydrogen gas comes from the water” being broken down by sunlight.
So water was present; sulfate and hydrogen could have as an energy source. But to survive, life as we know it needs one more ingredient carbon. Many living things obtain their carbon by breaking down the decayed remains of other dead organisms. But some, including several species of Desulfovibrio, are capable of creating organic material from scratch, as it were, drawing this critical ingredient of life directly from carbon dioxide (CO 2 ) gas. There’s plenty of that available on Mars.
All this gives reason to hope that life that found a way to exist on Mars back in the day when water was present. No one knows how long ago that was. Or whether such a time will come again. It may be that Mars dried up billions of years ago and has remained dry ever since. If that is the case, life is unlikely to have found a way to survive until the present.
Tilting toward Life
But Mars goes through cycles of obliquity, or changes in its orbital tilt. Currently, Mars is wobbling back and forth between 15 and 35 degrees’ obliquity, on a timescale of about 100,000 years. But every million years or so, it leans over as much as 60 degrees. Along with these changes in obliquity come changes in climate and atmosphere. Some scientists speculate that during the extremes of these obliquity cycles, Mars may develop an atmosphere as thick as Earth’s, and could warm up considerably. Enough for dormant life to reawaken.
“Because the climate can change on long terms,” says Clark, ice in some regions on Mars periodically could “become liquid enough that you would be able to actually come to life and do some things – grow, multiply, and so forth – and then go back to sleep again” when the thaw cycle ended. There are organisms on Earth that, when conditions become unfavorable, can form “spores which are so resistant that they can last for a very long time. Some people think millions of years, but that’s a little controversial.”
Desulfovibrio is not such an organism. It doesn’t form spores. But its bacterial cousin, Desulfotomaculum, does. “Usually the spores form because there’s something missing, like, for example, if hydrogen’s not available, or if there’s too much [oxygen], or if there’s not sulfate. The bacteria senses that the food source is going away, and it says, ‘I’ve got to hibernate,’ and will form the spores. The spores will stay dormant for extremely long periods of time. But they still have enough machinery operative that they can actually sense that nutrients are available. And then they’ll reconvert again in just a matter of hours, if necessary, to a living, breathing bacterium, so to speak. It’s pretty amazing,” says Clark.
That is not to say that future Mars landers should arrive with life-detection equipment tuned to zero in on species of Desulfovibrio or Desulfotomaculum. There is no reason to believe that life on Mars, if it ever emerged, evolved along the same lines as life on Earth, let alone that identical species appeared on the two planets. Still, the capabilities of various organisms on Earth indicate that life on Mars – including dormant organisms that could spring to life again in another few hundred thousand years – is certainly possible.
Clark says that he doesn’t “know that there’s any organism on Earth that could really operate on Mars, but over a long period of time, as the martian environment kept changing, what you would expect is that whatever life had started out there would keep adapting to the environment as it changed.”
Detecting such organisms is another matter. Don’t look for it to happen any time soon. Spirit and Opportunity were not designed to search for signs of life, but rather to search for signs of habitability. They could be rolling over fields littered with microscopic organisms in deep sleep and they’d never know it. Even future rovers will have a tough time identifying the martian equivalent of dormant bacterial spores.
“The spores themselves are so inert,” Clark says, “it’s a question, if you find a spore, and you’re trying to detect life, how do you know it’s a spore, [and not] just a little particle of sand? And the answer is: You don’t. Unless you can find a way to make the spore do what’s called germinating, going back to the normal bacterial form.” That, however, is a challenge for another day.
Information
- Author Services
Initiatives
You are accessing a machine-readable page. In order to be human-readable, please install an RSS reader.
All articles published by MDPI are made immediately available worldwide under an open access license. No special permission is required to reuse all or part of the article published by MDPI, including figures and tables. For articles published under an open access Creative Common CC BY license, any part of the article may be reused without permission provided that the original article is clearly cited. For more information, please refer to https://www.mdpi.com/openaccess .
Feature papers represent the most advanced research with significant potential for high impact in the field. A Feature Paper should be a substantial original Article that involves several techniques or approaches, provides an outlook for future research directions and describes possible research applications.
Feature papers are submitted upon individual invitation or recommendation by the scientific editors and must receive positive feedback from the reviewers.
Editor’s Choice articles are based on recommendations by the scientific editors of MDPI journals from around the world. Editors select a small number of articles recently published in the journal that they believe will be particularly interesting to readers, or important in the respective research area. The aim is to provide a snapshot of some of the most exciting work published in the various research areas of the journal.
Original Submission Date Received: .
- Active Journals
- Find a Journal
- Proceedings Series
- For Authors
- For Reviewers
- For Editors
- For Librarians
- For Publishers
- For Societies
- For Conference Organizers
- Open Access Policy
- Institutional Open Access Program
- Special Issues Guidelines
- Editorial Process
- Research and Publication Ethics
- Article Processing Charges
- Testimonials
- Preprints.org
- SciProfiles
- Encyclopedia
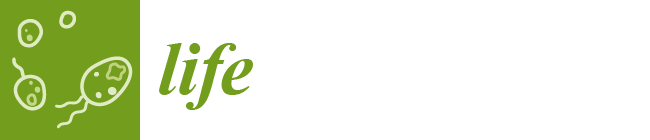
Article Menu
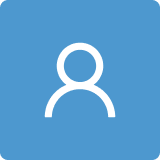
- Subscribe SciFeed
- Recommended Articles
- PubMed/Medline
- Google Scholar
- on Google Scholar
- Table of Contents
Find support for a specific problem in the support section of our website.
Please let us know what you think of our products and services.
Visit our dedicated information section to learn more about MDPI.
JSmol Viewer
Origin of life on mars: suitability and opportunities.
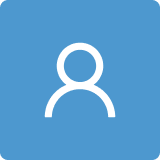
1. Introduction
2. materials and methods, 2.1. organic molecules and elements, 2.2. energy sources, 2.3. planetary settings, 3.1. elements and molecules on mars, 3.1.1. organics and chnops elements, 3.1.2. transition elements, 3.1.3. other key elements, 3.1.4. elements availability, 3.2. energy sources on mars, 3.3. settings on mars, 3.3.1. early mars as compared to early earth, 3.3.2. subaerial terrain proto-macrobionts, 3.3.3. suboceanic hydrothermal proto-macrobionts, 4. discussion, 4.1. when would be an ool on mars: past, present, future, 4.2. where an ool would have been on mars, 4.3. likelihood for origin of life, 4.3.1. expected value for the ool, 4.3.2. lithopanspermia, 4.4. future research, author contributions, institutional review board statement, informed consent statement, acknowledgments, conflicts of interest, abbreviations.
AmC | Amorphous Component (ChemCam XRD) |
APXS | Alpha Particle X-ray Spectrometer (XRF on MER, MSL rovers) |
a | Chemical activity of H O |
CCAM | ChemCam (MSL/LIBS on MSL rover) |
CheMin | Chemical and Mineralogy instrument (XRD on MSL rover) |
CRISM | Compact Recon Imaging Spectrometer for Mars (MRO orbiter) |
OMEGA | Vis/IR Mineralogical Mapping (ESA/Mars Express orbiter) |
EGA | Evolved Gas Analyzer (part of SAM on MSL rover) |
GHS | Geothermal Hot Spring |
GCMSGas | Chromatograph and Mass Spectrometer (Viking, MSL/SAM) |
GCRGalactic | Cosmic Radiation |
LAWKI | Life As We Know It (biochemically) |
LIBS | Laser-Induced Breakdown Spectroscopy |
MERMars | Exploration Rover (Spirit and Opportunity rovers) |
MORB | Mid-Ocean Ridge Basalt |
MS | Mass Spectrometer |
MSL | Mars Science Laboratory (Curiosity rover) |
M2020 | Mars 2020 Mission (Perseverance rover) |
NWA | Northwest Africa (meteorite identifier) |
OoL | Origin of Life |
OHV | Oceanic Hydrothermal Vent |
PAT | Pond in Ambient Temperature |
PIXL | Planetary Instrument for X-ray Lithochemistry (M2020 rover) |
PCE | Prebiotic chemical evolution |
RNAzyme | RNA enzyme (ribozyme) |
SAM | Sample Analysis at Mars (GCMS, EGA on MSL rover) |
THEMIS | Thermal Emission Imaging System (Odyssey orbiter) |
WCL | Wet Chemistry Laboratory (instrument on Phoenix lander) |
XRD | X-ray Diffraction |
XRF | X-ray Fluorescence |
- Pimentel, G.C.; Forney, P.B.; Herr, K.C. Evidence about hydrate and solid water in the Martian surface from the 1969 Mariner infrared spectrometer. J. Geophys. Res. 1974 , 79 , 1623–1634. [ Google Scholar ] [ CrossRef ]
- Milton, D.J. Water and processes of degradation in the Martian landscape. J. Geophys. Res. 1973 , 78 , 4037–4047. [ Google Scholar ] [ CrossRef ]
- Klein, H.P. The search for life on Mars: What we learned from Viking. J. Geophys. Res. Planets 1998 , 103 , 28463–284636. [ Google Scholar ] [ CrossRef ]
- Wordsworth, R.D. The climate of early Mars. Annu. Rev. Earth Planet. Sci. 2016 , 44 , 381–408. [ Google Scholar ] [ CrossRef ] [ Green Version ]
- Ehlmann, B.L.; Edwards, C.S. Mineralogy of the Martian surface. Annu. Rev. Earth Planet. Sci. 2014 , 42 , 291–315. [ Google Scholar ] [ CrossRef ] [ Green Version ]
- Rampe, E.B.; Blake, D.F.; Bristow, T.F.; Ming, D.W.; Vaniman, D.T.; Morris, R.V.; Achilles, C.N.; Chipera, S.J.; Morrison, S.M.; Tu, V.M.; et al. Mineralogy and geochemistry of sedimentary rocks and eolian sediments in Gale crater, Mars: A review after six Earth years of exploration with Curiosity. Geochemistry 2020 , 80 , 125605. [ Google Scholar ] [ CrossRef ]
- McLennan, S.M.; Grotzinger, J.P.; Hurowitz, J.A.; Tosca, N.J. The sedimentary cycle on early Mars. Annu. Rev. Earth Planet. Sci. 2019 , 47 , 91–118. [ Google Scholar ] [ CrossRef ] [ Green Version ]
- Grotzinger, J.P.; Sumner, D.Y.; Kah, L.C.; Stack, K.; Gupta, S.; Edgar, L.; Rubin, D.; Lewis, K.; Schieber, J.; Mangold, N.; et al. A habitable fluvio-lacustrine environment at Yellowknife Bay, Gale Crater, Mars. Science 2014 , 24 , 343. [ Google Scholar ] [ CrossRef ] [ PubMed ]
- Knoll, A.H.; Carr, M.; Clark, B.; Marais, D.J.D.; Farmer, J.D.; Fischer, W.W.; Grotzinger, J.P.; McLennan, S.M.; Malin, M.; Schröder, C.; et al. Astrobiological implications of Meridiani sediments. Earth Planet. Sci. Lett. 2005 , 240 , 179–189. [ Google Scholar ] [ CrossRef ] [ Green Version ]
- Benner, S.A.; Kim, H.J. The Case for a Martian Origin for Earth Life. In Proceedings of the Instruments, Methods, and Missions for Astrobiology XVII, San Diego, CA, USA, 28 September 2015; International Society for Optics and Photonics: Bellingham, WA, USA; Volume 9606, p. 96060C. [ Google Scholar ] [ CrossRef ]
- Fornaro, T.; Steele, A.; Brucato, J.R. Catalytic/protective properties of martian minerals and implications for possible origin of life on Mars. Life 2018 , 8 , 56. [ Google Scholar ] [ CrossRef ] [ Green Version ]
- Sasselov, D.D.; Grotzinger, J.P.; Sutherland, J.D. The origin of life as a planetary phenomenon. Sci. Adv. 2020 , 6 , eaax3419. [ Google Scholar ] [ CrossRef ] [ PubMed ] [ Green Version ]
- Clark, B.C.; Kolb, V.M. Macrobiont: Cradle for the origin of life and creation of a biosphere. Life 2020 , 10 , 278. [ Google Scholar ] [ CrossRef ] [ PubMed ]
- Mojarro, A.; Jin, L.; Szostak, J.W.; Head, J.W.; Zuber, M.T. In search of the RNA world on Mars. Geobiology 2021 , 19 , 307–321. [ Google Scholar ] [ CrossRef ] [ PubMed ]
- Damer, B.; Deamer, D. The hot spring hypothesis for an origin of life. Astrobiology 2020 , 20 , 429–452. [ Google Scholar ] [ CrossRef ] [ PubMed ] [ Green Version ]
- McKay, C.P. An origin of life on Mars. Cold Spring Harb. Perspect. Biol. 2010 , 2 , a003509. [ Google Scholar ] [ CrossRef ] [ Green Version ]
- Frenkel-Pinter, M.; Rajaei, V.; Glass, J.B.; Hud, N.V.; Williams, L.D. Water and life: The medium is the message. J. Mol. Evol. 2021 , 11 , 1–10. [ Google Scholar ] [ CrossRef ]
- Miller, S.L. A production of amino acids under possible primitive earth conditions. Science 1953 , 117 , 528–529. [ Google Scholar ] [ CrossRef ] [ Green Version ]
- de Duve, C. Clues from Present-Day Biology: The Thioester World. In The Molecular Origins of Life: Assembling Pieces of the Puzzle ; Brack, A., Ed.; Cambridge University Press: Cambridge, UK, 2000; pp. 219–236. [ Google Scholar ]
- Muchowska, K.B.; Varma, S.J.; Moran, J. Nonenzymatic metabolic reactions and life’s origins. Chem. Rev. 2020 , 120 , 7708–7744. [ Google Scholar ] [ CrossRef ]
- Chevallot-Beroux, E.; Gorges, J.; Moran, J. Energy conservation via thioesters in a non-enzymatic metabolism-like reaction network. ChemRxiv 2019 , 9 , 8832425. [ Google Scholar ]
- Goldford, J.E.; Hartman, H.; Marsland, R.; Segrè, D. Environmental boundary conditions for the origin of life converge to an organo-sulfur metabolism. Nat. Ecol. Evol. 2019 , 3 , 1715–1724. [ Google Scholar ] [ CrossRef ]
- Muchowska, K.B.; Moran, J. Peptide synthesis at the origin of life. Science 2020 , 370 , 767–768. [ Google Scholar ] [ CrossRef ] [ PubMed ]
- Wächtershäuser, G. The origin of life and its methodological challenge. J. Theor. Biol. 1997 , 187 , 483–494. [ Google Scholar ] [ CrossRef ] [ PubMed ]
- Wächtershäuser, G. From volcanic origins of chemoautotrophic life to Bacteria, Archaea and Eukarya. Philos. Trans. R. B Biol. Sci. 2006 , 361 , 1787–1808. [ Google Scholar ] [ CrossRef ] [ PubMed ] [ Green Version ]
- Wächtershäuser, G. On the chemistry and evolution of the pioneer organism. Chem. Biodivers. 2007 , 4 , 584–602. [ Google Scholar ] [ CrossRef ] [ PubMed ]
- Ragsdale, S.W.; Pierce, E. Acetogenesis and the Wood-Ljungdahl pathway of CO 2 fixation. Biochim. Biophys. Acta (BBA) Proteins Proteom. 2008 , 1784 , 1873–1898. [ Google Scholar ] [ CrossRef ] [ Green Version ]
- Todd, Z.R.; House, C.H. Vesicles protect activated acetic acid. Astrobiology 2014 , 14 , 859–865. [ Google Scholar ] [ CrossRef ]
- Russell, M.J.; Martin, W. The rocky roots of the acetyl-CoA pathway. Trends Biochem. Sci. 2004 , 29 , 358–363. [ Google Scholar ] [ CrossRef ] [ Green Version ]
- Ferry, J.G.; House, C.H. The stepwise evolution of early life driven by energy conservation. Mol. Biol. Evol. 2005 , 23 , 1286–1292. [ Google Scholar ] [ CrossRef ] [ Green Version ]
- Patel, B.H.; Percivalle, C.; Ritson, D.J.; Duffy, C.D.; Sutherland, J.D. Common origins of RNA, protein and lipid precursors in a cyanosulfidic protometabolism. Nat. Chem. 2015 , 7 , 301–307. [ Google Scholar ] [ CrossRef ] [ Green Version ]
- Liu, Z.; Wu, L.F.; Xu, J.; Bonfio, C.; Russell, D.A.; Sutherland, J.D. Harnessing chemical energy for the activation and joining of prebiotic building blocks. Nat. Chem. 2020 , 12 , 1–6. [ Google Scholar ] [ CrossRef ]
- Ritson, D.J.; Battilocchio, C.; Ley, S.V.; Sutherland, J.D. Mimicking the surface and prebiotic chemistry of early Earth using flow chemistry. Nat. Commun. 2018 , 9 , 1–10. [ Google Scholar ]
- Ferus, M.; Kubelík, P.; Knížek, A.; Pastorek, A.; Sutherland, J.; Civiš, S. High energy radical chemistry formation of HCN-rich atmospheres on early Earth. Sci. Rep. 2017 , 7 , 1–9. [ Google Scholar ] [ CrossRef ] [ Green Version ]
- Xu, J.; Ritson, D.J.; Ranjan, S.; Todd, Z.R.; Sasselov, D.D.; Sutherland, J.D. Photochemical reductive homologation of hydrogen cyanide using sulfite and ferrocyanide. Chem. Commun. 2018 , 54 , 5566–5569. [ Google Scholar ] [ CrossRef ]
- Toner, J.D.; Catling, D.C. Alkaline lake settings for concentrated prebiotic cyanide and the origin of life. Geochim. Cosmochim. Acta 2019 , 260 , 124–132. [ Google Scholar ] [ CrossRef ]
- Foden, C.S.; Islam, S.; Fernández-García, C.; Maugeri, L.; Sheppard, T.D.; Powner, M.W. Prebiotic synthesis of cysteine peptides that catalyze peptide ligation in neutral water. Science 2020 , 370 , 865–869. [ Google Scholar ] [ CrossRef ]
- Becker, S.; Feldmann, J.; Wiedemann, S.; Okamura, H.; Schneider, C.; Iwan, K.; Crisp, A.; Rossa, M.; Amatov, T.; Carell, T. Unified prebiotically plausible synthesis of pyrimidine and purine RNA ribonucleotides. Science 2019 , 366 , 76–82. [ Google Scholar ] [ CrossRef ] [ Green Version ]
- Becker, S.; Schneider, C.; Okamura, H.; Crisp, A.; Amatov, T.; Dejmek, M.; Carell, T. Wet-dry cycles enable the parallel origin of canonical and non-canonical nucleosides by continuous synthesis. Nat. Commun. 2018 , 9 , 1–9. [ Google Scholar ] [ CrossRef ] [ Green Version ]
- Becker, S.; Thoma, I.; Deutsch, A.; Gehrke, T.; Mayer, P.; Zipse, H.; Carell, T. A high-yielding, strictly regioselective prebiotic purine nucleoside formation pathway. Science 2016 , 352 , 833–836. [ Google Scholar ] [ CrossRef ]
- Becker, S.; Schneider, C.; Crisp, A.; Carell, T. Non-canonical nucleosides and chemistry of the emergence of life. Nat. Commun. 2018 , 9 , 1–4. [ Google Scholar ] [ CrossRef ]
- Silva, J.D.; Franco, A. Boron in prebiological evolution. Angew. Chem. 2020 , 133 , 10550–10560. [ Google Scholar ] [ CrossRef ]
- Ricardo, A.; Carrigan, M.A.; Olcott, A.N.; Benner, S.A. Borate minerals stabilize ribose. Science 2004 , 303 , 196. [ Google Scholar ] [ CrossRef ] [ Green Version ]
- Kim, H.J.; Furukawa, Y.; Kakegawa, T.; Bita, A.; Scorei, R.; Benner, S.A. Evaporite borate-containing mineral ensembles make phosphate available and regiospecifically phosphorylate ribonucleosides: Borate as a multifaceted problem solver in prebiotic chemistry. Angew. Chem. 2016 , 128 , 16048–16052. [ Google Scholar ] [ CrossRef ]
- Kim, H.J.; Ricardo, A.; Illangkoon, H.I.; Kim, M.J.; Carrigan, M.A.; Frye, F.; Benner, S.A. Synthesis of carbohydrates in mineral-guided prebiotic cycles. J. Am. Chem. Soc. 2011 , 133 , 9457–9468. [ Google Scholar ] [ CrossRef ]
- Kitadai, N.; Maruyama, S. Origins of building blocks of life: A review. Geosci. Front. 2018 , 9 , 1117–1153. [ Google Scholar ] [ CrossRef ]
- Kim, H.J.; Benner, S.A. Abiotic synthesis of nucleoside 5′-triphosphates with nickel borate and cyclic trimetaphosphate (CTMP). Astrobiology 2021 , 21 , 298–306. [ Google Scholar ] [ CrossRef ] [ PubMed ]
- Kawai, J.; McLendon, D.C.; Kim, H.J.; Benner, S.A. Hydroxymethanesulfonate from volcanic sulfur dioxide: A “mineral” reservoir for formaldehyde and other simple carbohydrates in prebiotic chemistry. Astrobiology 2019 , 19 , 506–516. [ Google Scholar ] [ CrossRef ] [ PubMed ] [ Green Version ]
- Ferris, J.P.; Hill, A.R.; Liu, R.; Orgel, L.E. Synthesis of long prebiotic oligomers on mineral surfaces. Nature 1996 , 381 , 59–61. [ Google Scholar ] [ CrossRef ] [ PubMed ]
- Huang, W.; Ferris, J.P. One-step, regioselective synthesis of up to 50-mers of RNA oligomers by montmorillonite catalysis. J. Am. Chem. Soc. 2006 , 128 , 8914–8919. [ Google Scholar ] [ CrossRef ]
- Varma, S.J.; Muchowska, K.B.; Chatelain, P.; Moran, J. Native iron reduces CO 2 to intermediates and end-products of the acetyl-CoA pathway. Nat. Ecol. Evol. 2018 , 2 , 1019–1024. [ Google Scholar ] [ CrossRef ]
- Martin, W.F. Older than genes: The acetyl CoA pathway and origins. Front. Microbiol. 2020 , 11 , 817. [ Google Scholar ] [ CrossRef ]
- Deamer, D.W. Membrane Compartments in Prebiotic Evolution. In The Molecular Origins of Life: Assembling Pieces of the Puzzle ; Brack, A., Ed.; Cambridge University Press: Cambridge, UK, 2000; pp. 189–205. [ Google Scholar ]
- Deamer, D.; Damer, B.; Kompanichenko, V. Hydrothermal chemistry and the origin of cellular life. Astrobiology 2019 , 19 , 1523–1537. [ Google Scholar ] [ CrossRef ] [ PubMed ]
- Cornell, C.E.; Black, R.A.; Xue, M.; Litz, H.E.; Ramsay, A.; Gordon, M.; Mileant, A.; Cohen, Z.R.; Williams, J.A.; Lee, K.K.; et al. Prebiotic amino acids bind to and stabilize prebiotic fatty acid membranes. Proc. Natl. Acad. Sci. USA 2019 , 116 , 17239–172344. [ Google Scholar ] [ CrossRef ] [ Green Version ]
- Jin, L.; Engelhart, A.E.; Zhang, W.; Adamala, K.; Szostak, J.W. Catalysis of template-directed nonenzymatic RNA copying by Iron (II). J. Am. Chem. Soc. 2018 , 140 , 15016–15021. [ Google Scholar ] [ CrossRef ] [ Green Version ]
- O’Flaherty, D.K.; Kamat, N.P.; Mirza, F.N.; Li, L.; Prywes, N.; Szostak, J.W. Copying of mixed-sequence RNA templates inside model protocells. J. Am. Chem. Soc. 2018 , 140 , 5171–5178. [ Google Scholar ] [ CrossRef ] [ Green Version ]
- Li, M.; Huang, X.; Tang, T.D.; Mann, S. Synthetic cellularity based on non-lipid micro-compartments and protocell models. Curr. Opin. Chem. Biol. 2014 , 22 , 1–11. [ Google Scholar ] [ CrossRef ]
- Deamer, D.; Singaram, S.; Rajamani, S.; Kompanichenko, V.; Guggenheim, S. Self-assembly processes in the prebiotic environment. Philos. Trans. R. Soc. B Biol. Sci. 2006 , 361 , 1809–1818. [ Google Scholar ] [ CrossRef ] [ Green Version ]
- Ross, D.S.; Deamer, D. Dry/wet cycling and the thermodynamics and kinetics of prebiotic polymer synthesis. Life 2016 , 6 , 28. [ Google Scholar ] [ CrossRef ] [ Green Version ]
- Milshteyn, D.; Damer, B.; Havig, J.; Deamer, D. Amphiphilic compounds assemble into membranous vesicles in hydrothermal hot spring water but not in seawater. Life 2018 , 8 , 11. [ Google Scholar ] [ CrossRef ] [ Green Version ]
- Tjhung, K.F.; Sczepanski, J.T.; Murtfeldt, E.R.; Joyce, G.F. RNA-catalyzed cross-chiral polymerization of RNA. J. Am. Chem. Soc. 2020 , 142 , 15331–15339. [ Google Scholar ] [ CrossRef ]
- Joyce, G.F.; Szostak, J.W. Protocells and RNA self-replication. Cold Spring Harb. Perspect. Biol. 2018 , 10 , a034801. [ Google Scholar ] [ CrossRef ] [ Green Version ]
- Horning, D.P.; Joyce, G.F. Amplification of RNA by an RNA polymerase ribozyme. Proc. Natl. Acad. Sci. USA 2016 , 113 , 9786–9791. [ Google Scholar ] [ CrossRef ] [ Green Version ]
- Joyce, G.F.; Orgel, L.E. Prospects for Understanding of RNA World. In The RNA World: The Nature of Modern RNA Suggests a Prebiotic RNA World ; Gesteland, R.F., Atkins, J.F., Eds.; Cold Spring Harbor University Press: Cold Spring Harbor, NY, USA, 1993; pp. 1–25. [ Google Scholar ]
- Robertson, M.P.; Joyce, G.F. The origins of the RNA World. Cold Spring Harb. Perspect. Biol. 2012 , 4 , a003608. [ Google Scholar ] [ CrossRef ]
- Tjhung, K.F.; Shokhirev, M.N.; Horning, D.P.; Joyce, G.F. An RNA polymerase ribozyme that synthesizes its own ancestor. Proc. Natl. Acad. Sci. USA 2020 , 117 , 2906–2913. [ Google Scholar ] [ CrossRef ]
- Kaim, W.; Schwederski, B.; Klein, A. Bioinorganic Chemistry—Inorganic Elements in the Chemistry of Life: An Introduction and Guide ; John Wiley & Sons: Hoboken, NJ, USA, 2013. [ Google Scholar ]
- Silva, J.F.D.; Williams, R.J. The Biological Chemistry of the Elements: The Inorganic Chemistry of Life ; Oxford University Press: Oxford, UK, 2001. [ Google Scholar ]
- Morowitz, H.J.; Srinivasan, V.; Smith, E. Ligand field theory and the origin of life as an emergent feature of the periodic table of elements. Biol. Bull. 2010 , 219 , 1–6. [ Google Scholar ] [ CrossRef ] [ PubMed ] [ Green Version ]
- Muchowska, K.B.; Varma, S.J.; Chevallot-Beroux, E.; Lethuillier-Karl, L.; Li, G.; Moran, J. Metals promote sequences of the reverse Krebs cycle. Nat. Ecol. Evol. 2017 , 1 , 1716–1721. [ Google Scholar ] [ CrossRef ]
- Degtyarenko, K. Bioinorganic motifs: Towards functional classification of metalloproteins. Bioinformatics 2000 , 16 , 851–864. [ Google Scholar ] [ CrossRef ] [ Green Version ]
- Hao, J.; Mokhtari, M.; Pedreira-Segade, U.; Michot, L.J.; Daniel, I. Transition metals enhance the adsorption of nucleotides onto clays: Implications for the origin of life. ACS Earth Space Chem. 2018 , 3 , 109–119. [ Google Scholar ] [ CrossRef ]
- Moore, E.K.; Jelen, B.I.; Giovannelli, D.; Raanan, H.; Falkowski, P.G. Metal availability and the expanding network of microbial metabolisms in the Archaean eon. Nat. Geosci. 2017 , 10 , 629–636. [ Google Scholar ] [ CrossRef ]
- Waldron, K.J.; Rutherford, J.C.; Ford, D.; Robinson, N.J. Metalloproteins and metal sensing. Nature 2009 , 460 , 823–830. [ Google Scholar ] [ CrossRef ]
- Frenkel-Pinter, M.; Samanta, M.; Ashkenasy, G.; Leman, L.J. Prebiotic peptides: Molecular hubs in the origin of life. Chem. Rev. 2020 , 11 , 4707–4765. [ Google Scholar ] [ CrossRef ] [ PubMed ]
- Van Der Gulik, P.; Massar, S.; Gilis, D.; Buhrman, H.; Rooman, M. The first peptides: The evolutionary transition between prebiotic amino acids and early proteins. J. Theor. Biol. 2009 , 261 , 531–539. [ Google Scholar ] [ CrossRef ] [ Green Version ]
- Presta, L.; Fondi, M.; Emiliani, G.; Fani, R. Molybdenum Availability in the Ecosystems (Geochemistry Aspects, When and How Did It Appear?). In Molybdenum Cofactors and Their role in the Evolution of Metabolic Pathways ; Springer: Dordrecht, The Netherlands, 2015. [ Google Scholar ] [ CrossRef ]
- Hickman-Lewis, K.; Cavalazzi, B.; Sorieul, S.; Gautret, P.; Foucher, F.; Whitehouse, M.J.; Jeon, H.; Georgelin, T.; Cockell, C.S.; Westall, F. Metallomics in deep time and the influence of ocean chemistry on the metabolic landscapes on Earth’s earliest ecosystems. Sci. Rep. 2020 , 10 , 1–16. [ Google Scholar ] [ CrossRef ] [ PubMed ]
- Mulkidjanian, A.Y.; Bychkov, A.Y.; Dibrova, D.V.; Galperin, M.Y.; Koonin, E.V. Origin of first cells at terrestrial, anoxic geothermal fields. Proc. Natl. Acad. Sci. USA 2012 , 109 , E821–E830. [ Google Scholar ] [ CrossRef ] [ Green Version ]
- Lu, G.S.; LaRowe, D.E.; Fike, D.A.; Druschel, G.K.; Gilhooly, W.P., III; Price, R.E.; Amend, J.P. Bioenergetic characterization of a shallow-sea hydrothermal vent system: Milos Island, Greece. PLoS ONE 2020 , 15 , e0234175. [ Google Scholar ] [ CrossRef ] [ PubMed ]
- Clark, B.C.; Kolb, V.M. Comet pond II: Synergistic intersection of concentrated extraterrestrial materials and planetary environments to form procreative Darwinian ponds. Life 2018 , 8 , 12. [ Google Scholar ] [ CrossRef ] [ Green Version ]
- Pearce, B.K.; Pudritz, R.E.; Ralph, E.; Semenov, D.A.; Henning, T.K. Origin of the RNA world: The fate of nucleobases in warm little ponds. Proc. Natl. Acad. Sci. USA 2017 , 114 , 11327–11332. [ Google Scholar ] [ CrossRef ] [ PubMed ] [ Green Version ]
- Baross, J.A. The rocky road to biomolecules. Nature 2018 , 564 , 42–43. [ Google Scholar ] [ CrossRef ] [ PubMed ]
- Baross, J.A.; Anderson, R.E.; Stüeken., E.E. The Environmental Roots of the Origin of Life. In Planetary Astrobiology ; Meadows, V.S., Arney, G.N., Schmidt, B.E., Marais, D.J.D., Eds.; University of Arizona: Tucson, AZ, USA, 2020; pp. 71–92. [ Google Scholar ]
- Westall, F.; Hickman-Lewis, K.; Hinman, N.; Gautret, P.; Campbell, K.A.; Bréhéret, J.G.; Foucher, F.; Hubert, A.; Sorieul, S.; Dass, A.V.; et al. A hydrothermal-sedimentary context for the origin of life. Astrobiology 2018 , 18 , 259–293. [ Google Scholar ] [ CrossRef ]
- Sojo, V.; Herschy, B.; Whicher, A.; Camprubi, E.; Lane, N. The origin of life in alkaline hydrothermal vents. Astrobiology 2016 , 16 , 181–197. [ Google Scholar ] [ CrossRef ] [ PubMed ]
- Egel, R. Primal eukaryogenesis: On the communal nature of precellular states, ancestral to modern life. Life 2012 , 2 , 170–212. [ Google Scholar ] [ CrossRef ] [ Green Version ]
- Russell, M.J. The alkaline solution to the emergence of life: Energy, entropy and early evolution. Acta Biotheor. 2007 , 55 , 133–179. [ Google Scholar ] [ CrossRef ] [ PubMed ]
- Russell, M.J.; Barge, L.M.; Bhartia, R.; Bocanegra, D.; Bracher, P.J.; Branscomb, E.; Kidd, R.; McGlynn, S.; Meier, D.H.; Nitschke, W.; et al. The drive to life on wet and icy worlds. Astrobiology 2014 , 14 , 308–343. [ Google Scholar ] [ CrossRef ]
- Russell, M.J.; Hall, A.J. The emergence of life from iron monosulphide bubbles at a submarine hydrothermal redox and pH front. J. Geol. Soc. 1997 , 154 , 377–402. [ Google Scholar ] [ CrossRef ] [ PubMed ] [ Green Version ]
- Maslennikov, V.V.; Maslennikova, S.P.; Large, R.R.; Danyushevsky, L.V.; Herrington, R.J.; Ayupova, N.R.; Zaykov, V.V.; Lein, A.Y.; Tseluyko, A.S.; Melekestseva, I.Y.; et al. Chimneys in Paleozoic massive sulfide mounds of the Urals VMS deposits: Mineral and trace element comparison with modern black, grey, white and clear smokers. Ore Geol. Rev. 2017 , 85 , 64–106. [ Google Scholar ] [ CrossRef ]
- Deamer, D.W. Assembling Life: How Can Life Begin on Earth and Other Habitable Planets? Oxford University Press: Oxford, UK, 2018. [ Google Scholar ]
- Chatterjee, S. A symbiotic view of the origin of life at hydrothermal impact crater-lakes. Phys. Chem. Chem. Phys. 2016 , 18 , 20033–20046. [ Google Scholar ] [ CrossRef ] [ PubMed ]
- Kring, D.A.; Whitehouse, M.J.; Schmieder, M. Microbial sulfur isotope fractionation in the Chicxulub hydrothermal system. Astrobiology 2020 , 21 , 103–114. [ Google Scholar ] [ CrossRef ]
- Osinski, G.R.; Cockell, C.S.; Pontefract, A.; Sapers, H.M. The role of meteorite impacts in the origin of life. Astrobiology 2020 , 20 , 1121–1149. [ Google Scholar ] [ CrossRef ] [ PubMed ]
- Benner, S.A.; Bell, E.A.; Biondi, E.; Brasser, R.; Carell, T.; Kim, H.J.; Trail, D. When did life likely emerge on Earth in an RNA-first process? Chem. Syst. Chem. 2019 , 2 , e1900035. [ Google Scholar ] [ CrossRef ]
- Lahav, N.; White, D.; Chang, S. Peptide formation in the prebiotic era: Thermal condensation of glycine in fluctuating clay environments. Science 1978 , 201 , 67–69. [ Google Scholar ] [ CrossRef ]
- Higgs, P.S. The effect of limited diffusion and wet-dry cycling on reversible polymerization reactions: Implications for prebiotic synthesis of nucleic acids. Life 2016 , 6 , 24. [ Google Scholar ] [ CrossRef ] [ Green Version ]
- Marais, D.J.D.; Walter, M.R. Terrestrial hot spring systems: Introduction. Astrobiology 2019 , 19 , 1419–1432. [ Google Scholar ] [ CrossRef ] [ PubMed ]
- Joshi, M.P.; Sawant, A.A.; Rajamani, S. Spontaneous emergence of membrane-forming protoamphiphiles from a lipid-amino acid mixture under wet-dry cycles. Chem. Sci. 2021 , 12 , 2970–2978. [ Google Scholar ] [ CrossRef ]
- Qiao, H.; Hu, N.; Bai, J.; Ren, L.; Liu, Q.; Fang, L.; Wang, Z. Encapsulation of nucleic acids into giant unilamellar vesicles by freeze-thaw: A way protocells may form. Orig. Life Evol. Biosph. 2017 , 47 , 499–510. [ Google Scholar ] [ CrossRef ]
- Vlassov, A.V.; Johnston, B.H.; Landweber, L.F.; Kazakov, S.A. Ligation activity of fragmented ribozymes in frozen solution: Implication for the RNAWorld. Nucleic Acids Res. 2004 , 32 , 2966–2974. [ Google Scholar ] [ CrossRef ] [ Green Version ]
- Mutschler, H.; Wochner, A.; Holliger, P. Freeze-thaw cycles as drivers of complex ribozyme assembly. Nat. Chem. 2015 , 7 , 502–508. [ Google Scholar ] [ CrossRef ] [ PubMed ] [ Green Version ]
- Feller, G. Cryosphere and psychrophiles: Insights into a cold origin of life? Life 2017 , 7 , 25. [ Google Scholar ] [ CrossRef ] [ Green Version ]
- Putnam, A.R.; Palucis, M.C. The hydrogeomorphic history of Garu crater: Implications and constraints on the timing of large late-stage lakes in the Gale crater region. J. Geophys. Res. Planets 2020 , e2020JE006688. [ Google Scholar ] [ CrossRef ]
- Salese, F.; Kleinhans, M.G.; Mangold, N.; Ansan, V.; McMahon, W.; Haas, T.D.; Dromart, G. Estimated minimum life span of the Jezero fluvial delta (Mars). Astrobiology 2020 , 20 , 977–993. [ Google Scholar ] [ CrossRef ]
- Schwartz, A.W. Evaluating the plausibility of prebiotic multistage syntheses. Astrobiology 2013 , 13 , 784–789. [ Google Scholar ] [ CrossRef ]
- Hunten, D.M. Possible oxidant sources in the atmosphere and surface of Mars. J. Mol. Evol. 1979 , 14 , 71–78. [ Google Scholar ] [ CrossRef ]
- Ranjan, S.; Todd, Z.R.; Sutherland, J.D.; Sasselov, D.D. Sulfidic anion concentrations on early Earth for surficial origins-of-life chemistry. Astrobiology 2018 , 18 , 1023–1040. [ Google Scholar ] [ CrossRef ] [ Green Version ]
- Achille, G.D.; Hynek, B.M. Ancient ocean on Mars supported by global distribution of deltas and valleys. Nat. Geosci. 2010 , 3 , 459–463. [ Google Scholar ] [ CrossRef ]
- Lasne, J.; Noblet, A.; Szopa, C.; Navarro-González, R.; Cabane, M.; Poch, O.; Stalport, F.; François, P.; Atreya, S.K.; Coll, P. Oxidants at the surface of Mars: A review in light of recent exploration results. Astrobiology 2016 , 16 , 977–996. [ Google Scholar ] [ CrossRef ] [ PubMed ] [ Green Version ]
- Ramirez, R.M.; Kopparapu, R.; Zugger, M.E.; Robinson, T.D.; Freedman, R.; Kasting, J.F. Warming early Mars with CO 2 and H 2 . Nat. Geosci. 2014 , 7 , 59–63. [ Google Scholar ] [ CrossRef ] [ Green Version ]
- Hayworth, B.P.; Kopparapu, R.K.; Haqq-Misra, J.; Batalha, N.E.; Payne, R.C.; Foley, B.J.; Ikwut-Ukwa, M.; Kasting, J.F. Warming early Mars with climate cycling: The effect of CO 2 -H 2 collision-induced absorption. Icarus 2020 , 27 , 113770. [ Google Scholar ] [ CrossRef ]
- Wordsworth, R.; Pierrehumbert, R. Hydrogen-nitrogen greenhouse warming in Earth’s early atmosphere. Science 2013 , 339 , 64–67. [ Google Scholar ] [ CrossRef ] [ Green Version ]
- Klein, F.; Tarnas, J.D.; Bach, W. Abiotic sources of molecular hydrogen on Earth. Elem. Int. Mag. Mineral. Geoch. Petrol. 2020 , 16 , 19–24. [ Google Scholar ] [ CrossRef ]
- Jakosky, B.M. The CO 2 inventory on Mars. Planet. Space Sci. 2019 , 175 , 52–59. [ Google Scholar ] [ CrossRef ]
- Kite, E.S.; Williams, J.P.; Lucas, A.; Aharonson, O. Low palaeopressure of the Martian atmosphere estimated from the size distribution of ancient craters. Nat. Geosci. 2014 , 7 , 335–339. [ Google Scholar ] [ CrossRef ] [ Green Version ]
- Warren, A.O.; Kite, E.S.; Williams, J.P.; Horgan, B. Through the thick and thin: New constraints on Mars paleopressure history 3.8–4 Ga from small exhumed craters. J. Geophys. Res. Planets. 2019 , 124 , 2793–2818. [ Google Scholar ] [ CrossRef ]
- Batalha, N.E.; Kopparapu, R.K.; Haqq-Misra, J.; Kasting, J.F. Climate cycling on early Mars caused by the carbonate–silicate cycle. Earth Planet. Sci. Lett. 2016 , 455 , 7–13. [ Google Scholar ] [ CrossRef ] [ Green Version ]
- Falkowski, P.; Scholes, R.J.; Boyle, E.E.; Canadell, J.; Canfield, D.; Elser, J.; Gruber, N.; Hibbard, K.; Högberg, P.; Linder, S.; et al. The global carbon cycle: A test of our knowledge of earth as a system. Science 2000 , 290 , 291–296. [ Google Scholar ] [ CrossRef ] [ PubMed ] [ Green Version ]
- Schmitt-Kopplin, P.; Harir, M.; Kanawati, B.; Gougeon, R.; Moritz, F.; Hertkorn, N.; Clary, S.; Gebefügi, I.; Gabelica, Z. Analysis of Extraterrestrial Organic Matter in Murchison Meteorite: A Progress Report. In Astrobiology: An Evolutionary Approach ; Kolb, V.M., Ed.; CRC Press/Taylor &Francis: Boca Raton, FL, USA, 2014; pp. 63–82. [ Google Scholar ]
- Yen, A.S.; Gellert, R.; Schröder, C.; Morris, R.V.; Bell, J.F.; Knudson, A.T.; Clark, B.C.; Ming, D.W.; Crisp, J.A.; Arvidson, R.E.; et al. An integrated view of the chemistry and mineralogy of Martian soils. Nature 2005 , 436 , 49–54. [ Google Scholar ] [ CrossRef ]
- Humayun, M.; Nemchin, A.; Zanda, B.; Hewins, R.H.; Grange, M.; Kennedy, A.; Lorand, J.P.; Göpel, C.; Fieni, C.; Pont, S.; et al. Origin and age of the earliest Martian crust from meteorite NWA 7533. Nature 2013 , 503 , 513–516. [ Google Scholar ] [ CrossRef ]
- Lodders, K. A survey of shergottite, nakhlite and chassigny meteorites whole-rock compositions. Meteorit. Planet. Sci. 1998 , 33 , A183–A190. [ Google Scholar ] [ CrossRef ]
- Airapetian, V.S.; Glocer, A.; Gronoff, G.; Hebrard, E.; Danchi, W. Prebiotic chemistry and atmospheric warming of early Earth by an active young Sun. Nat. Geosci. 2016 , 9 , 452–455. [ Google Scholar ] [ CrossRef ]
- Parkos, D.; Pikus, A.; Alexeenko, A.; Melosh, H.J. HCN production via impact ejecta reentry during the late heavy bombardment. J. Geophys. Res. Planets. 2018 , 123 , 892–909. [ Google Scholar ] [ CrossRef ]
- Matthews, C.N.; Minard, R.D. Hydrogen cyanide polymers, comets and the origin of life. Faraday Discuss. 2006 , 133 , 393–401. [ Google Scholar ] [ CrossRef ]
- Todd, Z.R.; Öberg, K.I. Cometary delivery of hydrogen cyanide to the early Earth. Astrobiology 2020 , 20 , 1109–1120. [ Google Scholar ] [ CrossRef ]
- Milo, R.; Phillips, R. Cell Biology by the Numbers ; Garland Science: New York, NY, USA, 2015. [ Google Scholar ]
- Ramirez, R.M. A warmer and wetter solution for early Mars and the challenges with transient warming. Icarus 2017 , 297 , 71–82. [ Google Scholar ] [ CrossRef ] [ Green Version ]
- Stern, J.C.; Sutter, B.; Freissinet, C.; Navarro-González, R.; McKay, C.P.; Archer, P.D.; Buch, A.; Brunner, A.E.; Coll, P.; Eigenbrode, J.L.; et al. Evidence for indigenous nitrogen in sedimentary and aeolian deposits from the Curiosity rover investigations at Gale crater, Mars. Proc. Natl. Acad. Sci. USA 2015 , 112 , 4245–4250. [ Google Scholar ] [ CrossRef ] [ Green Version ]
- Stern, J.C.; Sutter, B.; Jackson, W.A.; Navarro-González, R.; McKay, C.P.; Ming, D.W.; Archer, P.D.; Mahaffy, P.R. The nitrate/(per) chlorate relationship on Mars. Geophys. Res. Lett. 2017 , 44 , 2643–2651. [ Google Scholar ] [ CrossRef ] [ Green Version ]
- Ranjan, S.; Todd, Z.R.; Rimmer, P.B.; Sasselov, D.D.; Babbin, A.R. Nitrogen oxide concentrations in natural waters on early Earth. Geochem. Geophys. Geosyst. 2019 , 20 , 2021–2039. [ Google Scholar ] [ CrossRef ] [ Green Version ]
- Kounaves, S.P.; Hecht, M.H.; Kapit, J.; Quinn, R.C.; Catling, D.C.; Clark, B.C.; Ming, D.W.; Gospodinova, K.; Hredzak, P.; McElhoney, K.; et al. Soluble sulfate in the Martian soil at the Phoenix landing site. Geophys. Res. Lett. 2010 , 37 . [ Google Scholar ] [ CrossRef ] [ Green Version ]
- Stroble, S.T.; McElhoney, K.M.; Kounaves, S.P. Comparison of the Phoenix Mars lander WCL soil analyses with Antarctic Dry Valley soils, Mars meteorite EETA79001 sawdust, and a Mars simulant. Icarus 2013 , 225 , 933–939. [ Google Scholar ] [ CrossRef ]
- Steele, A.; Benning, L.G.; Wirth, R.; Siljeström, S.; Fries, M.D.; Hauri, E.; Conrad, P.G.; Rogers, K.; Eigenbrode, J.; Schreiber, A.; et al. Organic synthesis on Mars by electrochemical reduction of CO. Sci. Adv. 2018 , 4 , eaat5118. [ Google Scholar ] [ CrossRef ] [ Green Version ]
- Hu, L.; Khaniya, A.; Wang, J.; Chen, G.; Kaden, W.E.; Feng, X. Ambient electrochemical ammonia synthesis with high selectivity on Fe/Fe oxide catalyst. ACS Catal. 2018 , 8 , 9312–9319. [ Google Scholar ] [ CrossRef ]
- Du, H.; Yang, C.; Pu, W.; Zeng, L.; Gong, J. Enhanced electrochemical reduction of N 2 to ammonia over pyrite FeS 2 with excellent selectivity. ACS Sustain. Chem. Eng. 2020 , 8 , 10572–10580. [ Google Scholar ] [ CrossRef ]
- Fray, N.; Bardyn, A.; Cottin, H.; Baklouti, D.; Briois, C.; Engrand, C.; Fischer, H.; Hornung, K.; Isnard, R.; Langevin, Y.; et al. Nitrogen-to-carbon atomic ratio measured by COSIMA in the particles of comet 67P/Churyumov–Gerasimenko. Mon. Not. R. Astron. Soc. 2017 , 469 , S506–S516. [ Google Scholar ] [ CrossRef ] [ Green Version ]
- Clifford, S.M.; Parker, T.J. The evolution of the Martian hydrosphere: Implications for the fate of a primordial ocean and the current state of the northern plains. Icarus 2001 , 154 , 40–79. [ Google Scholar ] [ CrossRef ] [ Green Version ]
- Manning, C.V.; Zahnle, K.J.; McKay, C.P. Impact processing of nitrogen on early Mars. Icarus 2009 , 199 , 273–285. [ Google Scholar ] [ CrossRef ]
- Fox, J.L. The production and escape of nitrogen atoms on Mars. J. Geophys. Res. Planets. 1993 , 8 , 3297–3310. [ Google Scholar ] [ CrossRef ] [ Green Version ]
- McElroy, M.B.; Yung, Y.L.; Nier, A.O. Isotopic composition of nitrogen: Implications for the past history of Mars’ atmosphere. Science 1976 , 194 , 70–72. [ Google Scholar ] [ CrossRef ] [ Green Version ]
- Robinson, T.D.; Reinhard, C.T. Earth as an Exoplanet. In Planetary Astrobiology ; University of Arizona: Tucson, AZ, USA, 2020; pp. 379–416. [ Google Scholar ]
- Wordsworth, R.; Knoll, A.H.; Hurowitz, J.; Baum, M.; Ehlmann, B.L.; Head, J.W.; Steakley, K. A coupled model of episodic warming, oxidation and geochemical transitions on early Mars. Nat. Geosci. 2021 , 14 , 127–132. [ Google Scholar ] [ CrossRef ]
- Dreibus, G.; Jagoutz, E.; Spettel, B.; Wanke, H. Phosphate-mobilization on Mars? Implication from leach experiments on SNC’s. LPI 1996 , 27 , 323. [ Google Scholar ]
- Adcock, C.T.; Hausrath, E.M.; Forster, P.M. Readily available phosphate from minerals in early aqueous environments on Mars. Nat. Geosci. 2013 , 6 , 824–827. [ Google Scholar ] [ CrossRef ]
- Clark, B.C., III; Arvidson, R.E.; Gellert, R.; Morris, R.V.; Ming, D.W.; Richter, L.; Ruff, S.W.; Michalski, J.R.; Farrand, W.H.; Herkenhoff, K.E.; et al. Evidence for montmorillonite or its compositional equivalent in Columbia Hills, Mars. J. Geophys. Res. Planets 2007 , 112 . [ Google Scholar ] [ CrossRef ] [ Green Version ]
- Ming, D.W.; Mittlefehldt, D.W.; Morris, R.V.; Golden, D.C.; Gellert, R.; Yen, A.; Clark, B.C.; Squyres, S.W.; Farrand, W.H.; Ruff, S.W.; et al. Geochemical and mineralogical indicators for aqueous processes in the Columbia Hills of Gusev crater, Mars. J. Geophys. Res. Planets 2006 , 111 , E2. [ Google Scholar ] [ CrossRef ]
- McSween, H.Y.; Ruff, S.W.; Morris, R.V.; Bell, J.F., III; Herkenhoff, K.; Gellert, R.; Stockstill, K.R.; Tornabene, L.L.; Squyres, S.W.; Crisp, J.A.; et al. Alkaline volcanic rocks from the Columbia Hills, Gusev crater, Mars. J. Geophys. Res. Planets 2006 , 111 , E9. [ Google Scholar ] [ CrossRef ] [ Green Version ]
- McSween, H.Y.; Arvidson, R.E.; Bell, J.F.; Blaney, D.; Cabrol, N.A.; Christensen, P.R.; Clark, B.C.; Crisp, J.A.; Crumpler, L.S.; Marais, D.J.D.; et al. Basaltic rocks analyzed by the Spirit rover in Gusev Crater. Science 2004 , 305 , 842–845. [ Google Scholar ] [ CrossRef ] [ PubMed ]
- Berger, J.A.; Van Bommel, S.J.V.; Clark, B.C.; Gellert, R.; House, C.H.; King, P.L.; McCraig, M.A.; Ming, D.W.; O’Connell-Cooper, C.D.; Schmidt, M.E.; et al. Manganese and Phosphorus-Rich Nodules in Gale Crater, Mars: Results from the Groken Drill Site. In Proceedings of the 52nd Lunar and Planetary Science Conference, The Woodlands, TX, USA, 15–19 March 2021. [ Google Scholar ]
- Santos, A.R.; Agee, C.B.; McCubbin, F.M.; Shearer, C.K.; Burger, P.V.; Tartese, R.; Anand, M. Petrology of igneous clasts in Northwest Africa 7034: Implications for the petrologic diversity of the Martian crust. Geochim. Cosmochim. Acta 2015 , 157 , 56–85. [ Google Scholar ] [ CrossRef ]
- Liu, Y.; Fischer, W.W.; Ma, C.; Beckett, J.R.; Tschauner, O.; Guan, Y.; Lingappa, U.F.; Webb, S.M.; Prakapenka, V.B.; Lanza, N.L.; et al. Manganese oxides in Martian meteorites Northwest Africa (NWA) 7034 and 7533. Icarus 2021 , 364 , 114471. [ Google Scholar ] [ CrossRef ]
- Wellington, D.F.; Meslin, P.Y.; Van Beek, J.; Johnson, J.R.; Wiens, R.C.; Calef, F.J.; Bell, J.F. Iron Meteorite Finds Across Lower Mt. Sharp, Gale Crater, Mars: Clustering and Implications. In Proceedings of the 50th Lunar and Planetary Society Conference, The Woodlands, TX, USA, 18–22 March 2019. [ Google Scholar ]
- Fairen, A.G.; Dohm, J.M.; Baker, V.R.; Thompson, S.D.; Mahaney, W.C.; Herkenhoff, K.E.; Rodriguez, J.A.; Davila, A.F.; Schulze-makuch, D.; Maarry, M.R.E.; et al. Meteorites at Meridiani Planum provide evidence for significant amounts of surface and near-surface water on early Mars. Meteorit. Planet. Sci. 2011 , 46 , 1832–1841. [ Google Scholar ] [ CrossRef ]
- Meslin, P.Y.; Wellington, D.; Wiens, R.C.; Johnson, J.R.; Van Beek, J.; Gasnault, O.; Sautter, V.; Maroger, I.; Lasue, J.; Beck, P.; et al. Diversity and Areal Density of Iron-Nickel Meteorites Analyzed by Chemcam in Gale Crater. In Proceedings of the Lunar and Planetary Science Conference, The Woodlands, TX, USA, 18–22 March 2019; No. 2132; p. 3179. [ Google Scholar ]
- Lang, C.; Lago, J.; Pasek, M. Phosphorylation on the Early Earth: The Role of Phosphorus in Biochemistry and its Bioavailability. In Handbook of Astrobiology ; Kolb, V.M., Ed.; CRC Press: Boca Raton, FL, USA, 2019; pp. 361–370. [ Google Scholar ]
- Gibard, C.; Gorrell, I.B.; Jiménez, E.I.; Kee, T.P.; Pasek, M.A.; Krishnamurthy, R. Geochemical sources and availability of amidophosphates on the early Earth. Angew. Chem. 2019 , 131 , 8235–8239. [ Google Scholar ] [ CrossRef ] [ Green Version ]
- Burcar, B.; Castañeda, A.; Lago, J.; Daniel, M.; Pasek, M.A.; Hud, N.V.; Orlando, T.M.; Menor-Salván, C. A stark contrast to modern earth: Phosphate mineral transformation and nucleoside phosphorylation in an iron-and cyanide-rich early earth scenario. Angew. Chem. Int. Ed. 2019 , 58 , 16981–16987. [ Google Scholar ] [ CrossRef ]
- Clark, B.C.; Baird, A.K. Is the Martian lithosphere sulfur rich? J. Geophys. Res. Solid Earth 1979 , 84 , 8395–8403. [ Google Scholar ] [ CrossRef ]
- Franz, H.B.; King, P.L.; Gaillard, F. Sulfur on Mars from the Atmosphere to the Core. In Volatiles in the Martian Crust ; Elsevier: Amsterdam, The Netherlands, 2019; pp. 119–1483. [ Google Scholar ] [ CrossRef ]
- Sutherland, J.D. The origin of life—Out of the blue. Angew. Chem. Int. Ed. 2016 , 55 , 104–121. [ Google Scholar ] [ CrossRef ]
- Bonfio, C.; Valer, L.; Scintilla, S.; Shah, S.; Evans, D.J.; Jin, L.; Szostak, J.W.; Sasselov, D.D.; Sutherland, J.D.; Mansy, S.S. UV-light-driven prebiotic synthesis of iron–sulfur clusters. Nat. Chem. 2017 , 9 , 1229. [ Google Scholar ] [ CrossRef ] [ PubMed ]
- Xu, J.; Tsanakopoulou, M.; Magnani, C.J.; Szabla, R.; Šponer, J.E.; Šponer, J.; Góra, R.W.; Sutherland, J.D. A prebiotically plausible synthesis of pyrimidine β -ribonucleosides and their phosphate derivatives involving photoanomerization. Nat. Chem. 2017 , 9 , 303–309. [ Google Scholar ] [ CrossRef ] [ Green Version ]
- Styrt, M.M.; Brackmann, A.J.; Holland, H.D.; Clark, B.C.; Pisutha-Arnond, V.; Eldridge, C.S.; Ohmoto, H. The mineralogy and the isotopic composition of sulfur in hydrothermal sulfide/sulfate deposits on the East Pacific Rise, 21 N latitude. Earth Planet. Sci. Lett. 1981 , 53 , 382–390. [ Google Scholar ] [ CrossRef ]
- Gale, A.; Dalton, C.A.; Langmuir, C.H.; Su, Y.; Schilling, J.G. The mean composition of ocean ridge basalts. Geochem. Geophys. Geosyst. 2013 , 14 , 489–518. [ Google Scholar ] [ CrossRef ] [ Green Version ]
- Clark, B.C. Geochemical components in Martian soil. Geochim. Cosmochim. Acta 1993 , 57 , 4575–4581. [ Google Scholar ] [ CrossRef ]
- Yen, A.S.; Morris, R.V.; Clark, B.C.; Gellert, R.; Knudson, A.T.; Squyres, S.; Mittlefehldt, D.W.; Ming, D.W.; Arvidson, R.; McCoy, T.; et al. Hydrothermal processes at Gusev Crater: An evaluation of Paso Robles class soils. J. Geophys. Res. Planets 2008 , 113 , E6. [ Google Scholar ] [ CrossRef ] [ Green Version ]
- Clark, B.C.; Morris, R.V.; McLennan, S.M.; Gellert, R.; Jolliff, B.; Knoll, A.H.; Squyres, S.W.; Lowenstein, T.K.; Ming, D.W.; Tosca, N.J.; et al. Chemistry and mineralogy of outcrops at Meridiani Planum. Earth Planet. Sci. Lett. 2005 , 240 , 73–94. [ Google Scholar ] [ CrossRef ]
- Wang, A.; Haskin, L.A.; Squyres, S.W.; Jolliff, B.L.; Crumpler, L.; Gellert, R.; Schröder, C.; Herkenhoff, K.; Hurowitz, J.; Tosca, N.J.; et al. Sulfate deposition in subsurface regolith in Gusev crater, Mars. J. Geophys. Res. Planets 2006 , 111 , E2. [ Google Scholar ] [ CrossRef ] [ Green Version ]
- Rapin, W.; Ehlmann, B.L.; Dromart, G.; Schieber, J.; Thomas, N.H.; Fischer, W.W.; Fox, V.K.; Stein, N.T.; Nachon, M.; Clark, B.C.; et al. An interval of high salinity in ancient Gale crater lake on Mars. Nat. Geosci. 2019 , 12 , 889–895. [ Google Scholar ] [ CrossRef ] [ Green Version ]
- Yen, A.S.; Gellert, R.; Achilles, C.N.; Berger, J.A.; Blake, D.F.; Clark, B.C.; McAdam, C.; Wing, D.M.; Morris, R.V.; Morrison, S.M.; et al. Origin and Speciation of Sulfur Compounds in the Murray Formation, Gale Crater, Mars. In Proceedings of the Lunar and Planetary Science Conference, The Woodlands, TX, USA, 18–22 March 2019; Volume 50, p. 2083. [ Google Scholar ]
- Crisler, J.D.; Newville, T.M.; Chen, F.; Clark, B.C.; Schneegurt, M.A. Bacterial growth at the high concentrations of magnesium sulfate found in Martian soils. Astrobiology 2012 , 12 , 98–106. [ Google Scholar ] [ CrossRef ]
- Morris, R.V.; Schröder, C.; Klingelhöfer, G.; Agresti, D.G. Mössbauer Spectroscopy at Gusev Crater and Meridiani Planum: Iron Mineralogy, Oxidation State, and Alteration on Mars. In Remote Compositional Analysis: Techniques for Understanding Spectroscopy, Mineralogy, and Geochemistry of Planetary Surfaces ; Bishop, J.L., Bell, J.F., Moersch, J.E., Eds.; Cambridge Planetary Science, University Press: Cambridge, UK, 2019; pp. 538–554. [ Google Scholar ] [ CrossRef ]
- Squyres, S.W.; Arvidson, R.E.; Bell, J.F.; Calef, F.; Clark, B.C.; Cohen, B.A.; Crumpler, L.A.; Souza, P.A.D.; Farrand, W.H.; Gellert, R.; et al. Ancient impact and aqueous processes at Endeavour Crater, Mars. Science 2012 , 336 , 570–576. [ Google Scholar ] [ CrossRef ] [ Green Version ]
- Clark, B.C.; Van Hart, D.C. The salts of Mars. Icarus 1981 , 45 , 370–378. [ Google Scholar ] [ CrossRef ]
- Banin, A.; Han, F.X.; Kan, I.; Cicelsky, A. Acidic volatiles and the Mars soil. J. Geophys. Res. Planets 1997 , 102 , 13341–13356. [ Google Scholar ] [ CrossRef ]
- Settle, M. Formation and deposition of volcanic sulfate aerosols on Mars. J. Geophys. Res. Solid Earth 1979 , 84 , 8343–8354. [ Google Scholar ] [ CrossRef ]
- Quinn, R.C.; Chittenden, J.D.; Kounaves, S.P.; Hecht, M.H. The oxidation-reduction potential of aqueous soil solutions at the Mars Phoenix landing site. Geophys. Res. Lett. 2011 , 38 . [ Google Scholar ] [ CrossRef ]
- Bandfield, J.L.; Glotch, T.D.; Christensen, P.R. Spectroscopic identification of carbonate minerals in the Martian dust. Science 2003 , 301 , 1084–1087. [ Google Scholar ] [ CrossRef ] [ PubMed ] [ Green Version ]
- Wong, G.M.; Lewis, J.M.; Knudson, C.A.; Millan, M.; McAdam, A.C.; Eigenbrode, J.L.; Andrejkovičová, S.; Gómez, F.; Navarro-González, R.; House, C.H. Detection of reduced sulfur on Vera Rubin ridge by quadratic discriminant analysis of volatiles observed during evolved gas analysis. J. Geophys. Res. Planets 2020 , 125 , e2019JE006304. [ Google Scholar ] [ CrossRef ]
- Biemann, K.; Oro, J.I.; Toulmin, P., III; Orgel, L.E.; Nier, A.O.; Anderson, D.M.; Simmonds, P.G.; Flory, D.; Diaz, A.V.; Rushneck, D.R.; et al. The search for organic substances and inorganic volatile compounds in the surface of Mars. J. Geophys. Res. 1977 , 82 , 4641–4658. [ Google Scholar ] [ CrossRef ]
- Hubbard, J.S.; Hardy, J.P.; Horowitz, N.H. Photocatalytic production of organic compounds from CO and H 2 O in a simulated martian atmosphere. Proc. Natl. Acad. Sci. USA 1971 , 68 , 574–578. [ Google Scholar ] [ CrossRef ] [ Green Version ]
- Szopa, C.; Freissinet, C.; Glavin, D.P.; Millan, M.; Buch, A.; Franz, H.B.; Summons, R.E.; Sumner, D.Y.; Sutter, B.; Eigenbrode, J.L.; et al. First detections of dichlorobenzene isomers and trichloromethylpropane from organic matter indigenous to mars mudstone in gale crater, Mars: Results from the sample analysis at Mars instrument onboard the curiosity rover. Astrobiology 2020 , 20 , 292–306. [ Google Scholar ] [ CrossRef ]
- Eigenbrode, J.L.; Summons, R.E.; Steele, A.; Freissinet, C.; Millan, M.; Navarro-González, R.; Sutter, B.; McAdam, A.C.; Franz, H.B.; Glavin, D.P.; et al. Organic matter preserved in 3-billion-year-old mudstones at Gale crater, Mars. Science 2018 , 360 , 1096–1101. [ Google Scholar ] [ CrossRef ] [ Green Version ]
- Steele, A.; McCubbin, F.M.; Fries, M.; Kater, L.; Boctor, N.Z.; Fogel, M.L.; Conrad, P.G.; Glamoclija, M.; Spencer, M.; Morrow, A.L.; et al. A reduced organic carbon component in martian basalts. Science 2012 , 337 , 212–215. [ Google Scholar ] [ CrossRef ]
- Brown, P.G.; Hildebrand, A.R.; Zolensky, M.E.; Grady, M.; Clayton, R.N.; Mayeda, T.K.; Tagliaferri, E.; Spalding, R.; MacRae, N.D.; Hoffman, E.L.; et al. The fall, recovery, orbit, and composition of the Tagish Lake meteorite: A new type of carbonaceous chondrite. Science 2000 , 290 , 320–325. [ Google Scholar ] [ CrossRef ] [ Green Version ]
- Mustard, J.F.; Murchie, S.L.; Pelkey, S.M.; Ehlmann, B.L.; Milliken, R.E.; Grant, J.A.; Bibring, J.P.; Poulet, F.; Bishop, J.; Dobrea, E.N.; et al. Hydrated silicate minerals on Mars observed by the Mars Reconnaissance Orbiter CRISM instrument. Nature 2008 , 454 , 305–309. [ Google Scholar ] [ CrossRef ]
- Clark, B.C.; Morris, R.V.; Herkenhoff, K.E.; Farrand, W.H.; Gellert, R.; Jolliff, B.L.; Arvidson., R.E.; Squyres, S.W.; Mittlefehldt, D.W.; Ming, D.W.; et al. Esperance: Multiple episodes of aqueous alteration involving fracture fills and coatings at Matijevic Hill, Mars. Am. Mineral. 2016 , 101 , 1515–1526. [ Google Scholar ] [ CrossRef ]
- Ferris, J.P. Catalyzed RNA synthesis for the RNA world. In The Molecular Origins of Life: Assembling Pieces of the Puzzle ; Brack, A., Ed.; Cambridge University Press: Cambridge, UK, 2000; pp. 255–268. [ Google Scholar ]
- Ming, D.W.; Morris, R.V.; Clark, B.C. Aqueous Alteration on Mars. In The Martian Surface ; Bell, J., Ed.; Cambridge University Press: Cambridge, UK, 2008; Chapter 23; pp. 519–540. [ Google Scholar ]
- Yen, A.S.; Morris, R.V.; Ming, D.W.; Schwenzer, S.P.; Sutter, B.; Vaniman, D.T.; Treiman, A.H.; Gellert, R.; Achilles, C.N.; Berger, J.A.; et al. Formation of tridymite and evidence for a hydrothermal history at gale crater, Mars. J. Geophys. Res. Planets 2021 . [ Google Scholar ] [ CrossRef ]
- Goetz, W.; Wiens, R.C.; Dehouck, E.; Gasnault, O.; Lasue, J.; Payre, V.; Frydenvang, J.; Clark, B.; Clegg, S.M.; Forni, O.; et al. Tracking of Copper by the ChemCam Instrument in Gale Crater, Mars: Elevated Abundances in Glen Torridon. In Proceedings of the Lunar and Planetary Science Conference, The Woodlands, TX, USA, 18–22 March 2020; Volume 2326, p. 2974. [ Google Scholar ]
- Payré, V.; Fabre, C.; Sautter, V.; Cousin, A.; Mangold, N.; Deit, L.L.; Forni, O.; Goetz, W.; Wiens, R.C.; Gasnault, O.; et al. Copper enrichments in the Kimberley formation in Gale crater, Mars: Evidence for a Cu deposit at the source. Icarus 2019 , 321 , 736–751. [ Google Scholar ] [ CrossRef ] [ Green Version ]
- VanBommel, S.J.; Gellert, R.; Berger, J.A.; Yen, A.S.; Boyd, N.I. Mars science laboratory alpha particle X-ray spectrometer trace elements: Situational sensitivity to Co, Ni, Cu, Zn, Ga, Ge, and Br. Acta Astronaut. 2019 , 165 , 32–42. [ Google Scholar ] [ CrossRef ]
- Gasda, P.J.; Haldeman, E.B.; Wiens, R.C.; Rapin, W.; Bristow, T.F.; Bridges, J.C.; Schwenzer, S.P.; Clark, B.; Herkenhoff, K.; Frydenvang, J.; et al. In situ detection of boron by ChemCam on Mars. Geophys. Res. Lett. 2017 , 44 , 8739–8748. [ Google Scholar ] [ CrossRef ]
- Lanza, N.L.; Fischer, W.W.; Wiens, R.C.; Grotzinger, J.; Ollila, A.M.; Cousin, A.; Anderson, R.B.; Clark, B.C.; Gellert, R.; Mangold, N.; et al. High manganese concentrations in rocks at Gale crater, Mars. Geophys. Res. Lett. 2014 , 41 , 5755–5763. [ Google Scholar ] [ CrossRef ] [ Green Version ]
- Lanza, N.L.; Wiens, R.C.; Arvidson, R.E.; Clark, B.C.; Fischer, W.W.; Gellert, R.; Grotzinger, J.P.; Hurowitz, J.A.; McLennan, S.M.; Morris, R.V.; et al. Oxidation of manganese in an ancient aquifer, Kimberley formation, Gale crater, Mars. Geophys. Res. Lett. 2016 , 43 , 7398–73407. [ Google Scholar ] [ CrossRef ] [ Green Version ]
- Arvidson, R.E.; Squyres, S.W.; Morris, R.V.; Knoll, A.H.; Gellert, R.; Clark, B.C.; Catalano, J.G.; Jolliff, B.L.; McLennan, S.M.; Herkenhoff, K.E.; et al. High concentrations of manganese and sulfur in deposits on Murray Ridge, Endeavour Crater, Mars. Am. Mineral. 2016 , 101 , 1389–1405. [ Google Scholar ] [ CrossRef ] [ Green Version ]
- Noda, N.; Imamura, S.; Sekine, Y.; Kurisu, M.; Fukushi, K.; Terada, N.; Uesugi, S.; Numako, C.; Takahashi, Y.; Hartmann, J. Highly oxidizing aqueous environments on early Mars inferred from scavenging pattern of trace metals on manganese oxides. J. Geophys. Res. Planets 2019 , 124 , 1282–1295. [ Google Scholar ] [ CrossRef ]
- Berger, J.A.; King, P.L.; Gellert, R.; Clark, B.C.; O’Connell-Cooper, C.D.; Thompson, L.M.; Van Bommel, S.J.; Yen, A.S. Manganese enrichment pathways relevant to Gale crater, Mars: Evaporative concentration and chlorine-induced precipitation. In Proceedings of the Lunar and Planetary Science Conference, The Woodlands, TX, USA, 18–22 March 2019; Volume 50, p. 2487. [ Google Scholar ]
- Salminen, R.; Batista, M.J.; Bidovec, M.; Demetriades, A.; Vivo, B.; Vos, W.D.; Duris, M.; Gilucis, A.; Gregorauskiene, V.; Halamić, J.; et al. Geochemical Atlas of Europe ; Part 1, Background Information, Methodology and Maps; Geological Survey of Finland: Espoo, Finland, 2005. [ Google Scholar ]
- Marshall, C.P.; Marshall, A.O.; Aitken, J.B.; Lai, B.; Vogt, S.; Breuer, P.; Steemans, P.; Lay, P.A. Imaging of vanadium in microfossils: A new potential biosignature. Astrobiology 2017 , 17 , 1069–1076. [ Google Scholar ] [ CrossRef ] [ PubMed ]
- Carpentier, W.; Sandra, K.; Smet, I.; Brige, A.; Smet, L.D.; Van Beeumen, J. Microbial reduction and precipitation of vanadium by Shewanella oneidensis . Appl. Environ. Microbiol. 2003 , 69 , 3636–3639. [ Google Scholar ] [ CrossRef ] [ PubMed ] [ Green Version ]
- Jochum, K.P.; Weis, U.; Schwager, B.; Stoll, B.; Wilson, S.A.; Haug, G.H.; Andreae, M.O.; Enzweiler, J. Reference values following ISO guidelines for frequently requested rock reference materials. Geostand. Geoanalytical Res. 2015 , 40 , 333–350. [ Google Scholar ] [ CrossRef ]
- Campbell, T.D.; Febrian, R.; McCarthy, J.T.; Kleinschmidt, H.E.; Forsythe, J.G.; Bracher, P.J. Prebiotic condensation through wet–dry cycling regulated by deliquescence. Nat. Commun. 2019 , 10 , 1–7. [ Google Scholar ] [ CrossRef ]
- Morris, R.V.; Rampe, E.B.; Vaniman, D.T.; Christoffersen, R.; Yen, A.S.; Morrison, S.M.; Ming, D.W.; Achilles, C.N.; Fraeman, A.A.; Le, L.; et al. Hydrothermal precipitation of sanidine (adularia) having full Al, Si structural disorder and specular hematite at Maunakea volcano (Hawai’i) and at Gale Crater (Mars). J. Geophys. Res. Planets 2020 , 125 , e2019JE006324. [ Google Scholar ] [ CrossRef ]
- Cousin, M.; Desjardins, E.; Dehouck, O.; Forni, G.; David, G.; Berger, G.; Caravaca, P.; Meslin, J.; Lasue, A.; Ollila, W.; et al. Wiens5, K-Rich Rubbly Bedrock at Glen Torridon, Gale Crater, Mars: Investigating the Possible Presence of Illite. In Proceedings of the 52nd Lunar and Planetary Science Conference 2021, The Woodlands, TX, USA, 15–19 March 2021. [ Google Scholar ]
- Bowman, J.C.; Petrov, A.S.; Frenkel-Pinter, M.; Penev, P.I.; Williams, L.D. Root of the tree: The significance, evolution, and origins of the ribosome. Chem. Rev. 2020 , 120 , 4848–4878. [ Google Scholar ] [ CrossRef ]
- Bray, M.S.; Lenz, T.K.; Haynes, J.W.; Bowman, J.C.; Petrov, A.S.; Reddi, A.R.; Hud, N.V.; Williams, L.D.; Glass, J.B. Multiple prebiotic metals mediate translation. Proc. Natl. Acad. Sci. USA 2018 , 115 , 12164–12169. [ Google Scholar ] [ CrossRef ] [ Green Version ]
- Kounaves, S.P.; Oberlin, E.A. Volatiles Measured by the Phoenix Lander at the Northern Plains of Mars. In Volatiles in the Martian Crust ; Elsevier: Amsterdam, The Netherlands, 2019; pp. 265–283. [ Google Scholar ] [ CrossRef ]
- Bibring, J.P.; Langevin, Y.; Mustard, J.F.; Poulet, F.; Arvidson, R.; Gendrin, A.; Gondet, B.; Mangold, N.; Pinet, P.; Forget, F.; et al. Global mineralogical and aqueous Mars history derived from OMEGA/Mars Express data. Science 2006 , 312 , 400–404. [ Google Scholar ] [ CrossRef ] [ Green Version ]
- Das, D.; Gasda, P.J.; Wiens, R.C.; Berlo, K.; Leveille, R.J.; Frydenvang, J.; Mangold, N.; Kronyak, R.E.; Schwenzer, S.P.; Forni, O.; et al. Boron and lithium in calcium sulfate veins: Tracking precipitation of diagenetic materials in Vera Rubin Ridge, Gale crater. J. Geophys. Res. Planets 2020 , 125 , e2019JE006301. [ Google Scholar ] [ CrossRef ]
- Stephenson, J.D.; Hallis, L.J.; Nagashima, K.; Freeland, S.J. Boron enrichment in martian clay. PLoS ONE 2013 , 8 , e64624. [ Google Scholar ] [ CrossRef ]
- Palmer, M.R.; Sturchio, N.C. The boron isotope systematics of the Yellowstone National Park (Wyoming) hydrothermal system: A reconnaissance. Geochim. Cosmochim. Acta 1990 , 54 , 2811–2815. [ Google Scholar ] [ CrossRef ]
- Anbar, A.D. Elements and evolution. Science 2008 , 5 , 1481–1483. [ Google Scholar ] [ CrossRef ]
- Chemtob, S.M.; Nickerson, R.D.; Morris, R.V.; Agresti, D.G.; Catalano, J.G. Oxidative alteration of ferrous smectites and implications for the redox evolution of early Mars. J. Geophys. Res. Planets 2017 , 122 , 2469–2488. [ Google Scholar ] [ CrossRef ] [ PubMed ] [ Green Version ]
- Bullock, M.A.; Moore, J.M. Atmospheric conditions on early Mars and the missing layered carbonates. Geophys. Res. Lett. 2007 , 34 . [ Google Scholar ] [ CrossRef ]
- Baird, A.K.; Clark, B.C. On the original igneous source of Martian fines. Icarus 1981 , 45 , 113–123. [ Google Scholar ] [ CrossRef ] [ Green Version ]
- Zolotov, M.Y.; Mironenko, M.V. Timing of acid weathering on Mars: A kinetic-thermodynamic assessment. J. Geophys. Res. Planets 2007 , 112 , E7. [ Google Scholar ] [ CrossRef ] [ Green Version ]
- Thomas, N.H.; Ehlmann, B.L.; Meslin, P.Y.; Rapin, W.; Anderson, D.E.; Rivera-Hernández, F.; Forni, O.; Schröder, S.; Cousin, A.; Mangold, N.; et al. Mars science laboratory observations of chloride salts in Gale crater, Mars. Geophys. Res. Lett. 2019 , 46 , 10754–10763. [ Google Scholar ] [ CrossRef ] [ Green Version ]
- Smith, R.J.; McLennan, S.M.; Achilles, C.N.; Dehouck, E.; Horgan, B.H.; Mangold, N.; Rampe, E.B.; Salvatore, M.; Siebach, K.L.; Sun, V. X-ray amorphous components in sedimentary rocks of Gale crater, Mars: Evidence for ancient formation and long-lived aqueous activity. J. Geophys. Res. Planets 2021 , 126 , e2020JE006782. [ Google Scholar ] [ CrossRef ]
- Jackson, R.S.; Wiens, R.C.; Vaniman, D.T.; Beegle, L.; Gasnault, O.; Newsom, H.E.; Maurice, S.; Meslin, P.Y.; Clegg, S.; Cousin, A.; et al. ChemCam investigation of the John Klein and Cumberland drill holes and tailings, Gale crater, Mars. Icarus 2016 , 277 , 330–341. [ Google Scholar ] [ CrossRef ]
- Yoshimura, Y. The Search for Life on Mars. In Astrobiology ; Yamagishi, A., Kakegawa, T., Usui, T., Eds.; Springer: Singapore, 2019; pp. 367–381. [ Google Scholar ] [ CrossRef ]
- Sholes, S.F.; Krissansen-Totton, J.; Catling, D.C. A maximum subsurface biomass on Mars from untapped free energy: CO and H 2 as potential antibiosignatures. Astrobiology 2019 , 19 , 655–668. [ Google Scholar ] [ CrossRef ] [ Green Version ]
- Price, A.; Pearson, V.K.; Schwenzer, S.P.; Miot, J.; Olsson-Francis, K. Nitrate-dependent iron oxidation: A potential Mars metabolism. Front. Microbiol. 2018 , 9 , 513. [ Google Scholar ] [ CrossRef ] [ PubMed ] [ Green Version ]
- Gonzalez-Toril, E.; Martínez-Frías, J.; Gomez, J.M.; Rull, F.; Amils, R. Iron meteorites can support the growth of acidophilic chemolithoauthrophic microorganisms. Astrobiology 2005 , 5 , 406–414. [ Google Scholar ] [ CrossRef ] [ PubMed ] [ Green Version ]
- Soare, R.; Conway, S.; Williams, J.-P.; Oehler, D. (Eds.) Mars Geological Enigmas: From the Late Noachian Epoch to the Present Day , 1st ed.; Elsevier: Amsterdam, The Netherlands, 2021; ISBN 9780128202456. [ Google Scholar ]
- Ernst, W.G. Earth’s thermal evolution, mantle convection, and Hadean onset of plate tectonics. J. Asian Earth Sci. 2017 , 145 , 334–348. [ Google Scholar ] [ CrossRef ]
- Faltys, J.P.; Wielicki, M.M. Inclusions in impact-formed zircon as a tracer of target rock lithology: Implications for Hadean continental crust composition and abundance. Lithos 2020 , 376 , 105761. [ Google Scholar ] [ CrossRef ]
- Bada, J.L.; Korenaga, J. Exposed areas above sea level on Earth >3.5 Gyr ago: Implications for prebiotic and primitive biotic chemistry. Life 2018 , 8 , 55. [ Google Scholar ] [ CrossRef ] [ Green Version ]
- Marshall, M. The water paradox and the origins of life. Nature 2020 , 588 , 210–213. [ Google Scholar ] [ CrossRef ]
- Carr, M.H.; Head, J.W. Martian surface/near-surface water inventory: Sources, sinks, and changes with time. Geophys. Res. Lett. 2015 , 42 , 726–732. [ Google Scholar ] [ CrossRef ]
- Palumbo, A.M.; Head, J.W.; Wordsworth, R.D. Late Noachian Icy Highlands climate model: Exploring the possibility of transient melting and fluvial/lacustrine activity through peak annual and seasonal temperatures. Icarus 2018 , 300 , 261–286. [ Google Scholar ] [ CrossRef ]
- Morris, R.V.; Klingelhoefer, G.; Schröder, C.; Fleischer, I.; Ming, D.W.; Yen, A.S.; Gellert, R.; Arvidson, R.E.; Rodionov, D.S.; Crumpler, L.S.; et al. Iron mineralogy and aqueous alteration from Husband Hill through Home Plate at Gusev crater, Mars: Results from the Mössbauer instrument on the Spirit Mars Exploration Rover. J. Geophys. Res. Planets 2008 , 113 , E12. [ Google Scholar ] [ CrossRef ]
- Tosca, N.J.; Ahmed, I.A.; Tutolo, B.M.; Ashpitel, A.; Hurowitz, J.A. Magnetite authigenesis and the warming of early Mars. Nat. Geosci. 2018 , 11 , 635–639. [ Google Scholar ] [ CrossRef ]
- Robbins, S.J.; Hynek, B.M. The secondary crater population of Mars. Earth Planet. Sci. Lett. 2014 , 400 , 66–76. [ Google Scholar ] [ CrossRef ]
- Segura, T.L.; Toon, O.B.; Colaprete, A. Modeling the environmental effects of moderate-sized impacts on Mars. J. Geophys. Res. Planets 2008 , 113 , E11. [ Google Scholar ] [ CrossRef ]
- Turbet, M.; Forget, F.; Head, J.W.; Wordsworth, R. 3D modelling of the climatic impact of outflow channel formation events on early Mars. Icarus 2017 , 288 , 10–36. [ Google Scholar ] [ CrossRef ] [ Green Version ]
- Haberle, R.M.; Zahnle, K.; Barlow, N.G.; Steakley, K.E. Impact degassing of H 2 on early Mars and its effect on the climate system. Geophys. Res. Lett. 2019 , 46 , 13355–13362. [ Google Scholar ] [ CrossRef ] [ Green Version ]
- Treiman, A.H.; Bish, D.L.; Vaniman, D.T.; Chipera, S.J.; Blake, D.F.; Ming, D.W.; Morris, R.V.; Bristow, T.F.; Morrison, S.M.; Baker, M.B.; et al. Mineralogy, provenance, and diagenesis of a potassic basaltic sandstone on Mars: CheMin X-ray diffraction of the Windjana sample (Kimberley area, Gale Crater). J. Geophys. Res. Planets 2016 , 121 , 75–106. [ Google Scholar ] [ CrossRef ] [ Green Version ]
- Fraeman, A.A.; Edgar, L.A.; Rampe, E.B.; Thompson, L.M.; Frydenvang, J.; Fedo, C.M.; Catalano, J.G.; Dietrich, W.E.; Gabriel, T.S.; Vasavada, A.R.; et al. Evidence for a diagenetic origin of Vera Rubin ridge, Gale crater, Mars: Summary and synthesis of Curiosity’s exploration campaign. J. Geophys. Res. Planets 2020 , 125 , e2020JE006527. [ Google Scholar ] [ CrossRef ] [ PubMed ]
- David, G.; Cousin, A.; Forni, O.; Meslin, P.Y.; Dehouck, E.; Mangold, N.; L’Haridon, J.; Rapin, W.; Gasnault, O.; Johnson, J.R.; et al. Analyses of high-iron sedimentary bedrock and diagenetic features observed with ChemCam at Vera Rubin ridge, Gale crater, Mars: Calibration and characterization. J. Geophys. Res. Planets 2020 , 125 , e2019JE006314. [ Google Scholar ] [ CrossRef ]
- Achilles, C.N.; Rampe, E.B.; Downs, R.T.; Bristow, T.F.; Ming, D.W.; Morris, R.V.; Vaniman, D.T.; Blake, D.F.; Yen, A.S.; McAdam, A.C.; et al. Evidence for multiple diagenetic episodes in ancient fluvial-lacustrine sedimentary rocks in Gale crater, Mars. J. Geophys. Res. Planets 2020 , 125 , e2019JE006295. [ Google Scholar ] [ CrossRef ] [ PubMed ]
- Craddock, R.A.; Lorenz, R.D. The changing nature of rainfall during the early history of Mars. Icarus 2017 , 293 , 172–179. [ Google Scholar ] [ CrossRef ]
- Quay, G.S.D.; Goudge, T.A.; Fassett, C.I. Precipitation and aridity constraints from paleolakes on early Mars. Geology 2020 , 48 , 1189–1193. [ Google Scholar ] [ CrossRef ]
- Palumbo, A.M.; Head, J.W.; Wilson, L. Rainfall on Noachian Mars: Nature, timing, and influence on geologic processes and climate history. Icarus 2020 , 11 , 113782. [ Google Scholar ] [ CrossRef ]
- Duran, S.; Coulthard, T.J. The Kasei Valles, Mars: A unified record of episodic channel flows and ancient ocean levels. Sci. Rep. 2020 , 10 , 1–7. [ Google Scholar ] [ CrossRef ] [ PubMed ]
- Soare, R.J.; Osinski, G.R.; Roehm, C.L. Thermokarst lakes and ponds on Mars in the very recent (late Amazonian) past. Earth Planet. Sci. Lett. 2008 , 272 , 382–393. [ Google Scholar ] [ CrossRef ]
- Grant, J.A.; Wilson, S.A.; Mangold, N.; Calef III, F. and Grotzinger, J.P. The timing of alluvial activity in Gale crater, Mars. Geophysical Res. Lett. 2014 , 41 , 1142–1149. [ Google Scholar ] [ CrossRef ] [ Green Version ]
- Comte, J.; Monier, A.; Crevecoeur, S.; Lovejoy, C.; Vincent, W.F. Microbial biogeography of permafrost thaw ponds across the changing northern landscape. Ecography 2016 , 39 , 609–618. [ Google Scholar ] [ CrossRef ]
- Kadoya, S.; Krissansen-Totton, J.; Catling, D.C. Probable cold and alkaline surface environment of the Hadean Earth caused by impact ejecta weathering. Geochem. Geophys. Syst. 2019 , 21 , e2019GC008734. [ Google Scholar ] [ CrossRef ] [ Green Version ]
- Buhler, P.B.; Piqueux, S. Mars Obliquity-Driven CO 2 Exchange between the Atmosphere, Regolith, and Polar Cap. J. Geophy. Res. Planets 2021 , e2020JE006759. [ Google Scholar ] [ CrossRef ]
- Knoll, A.H.; Jolliff, B.L.; Farrand, W.H.; Bell, I.I.I.J.F.; Clark, B.C.; Gellert, R.; Golombek, M.P.; Grotzinger, J.P.; Herkenhoff, K.E.; Johnson, J.R.; et al. Veneers, rinds, and fracture fills: Relatively late alteration of sedimentary rocks at Meridiani Planum, Mars. J. Geophys. Res. Planets 2008 , 113 , E6. [ Google Scholar ] [ CrossRef ] [ Green Version ]
- Osterloo, M.M.; Hamilton, V.E.; Bandfield, J.L.; Glotch, T.D.; Baldridge, A.M.; Christensen, P.R.; Tornabene, L.L.; Anderson, F.S. Chloride-bearing materials in the southern highlands of Mars. Science 2008 , 319 , 1651–1654. [ Google Scholar ] [ CrossRef ] [ Green Version ]
- Hynek, B.M.; Osterloo, M.K.; Kierein-Young, K.S. Late-stage formation of Martian chloride salts through ponding and evaporation. Geology 2015 , 43 , 787–790. [ Google Scholar ] [ CrossRef ] [ Green Version ]
- Rummel, J.D.; Beaty, D.W.; Jones, M.A.; Bakermans, C.; Barlow, N.G.; Boston, P.J.; Chevrier, V.F.; Clark, B.C.; Vera, J.P.D.; Gough, R.V.; et al. A new analysis of Mars “special regions”: Findings of the second MEPAG Special Regions Science Analysis Group (SR-SAG2). Astrobiology 2014 , 14 , 887–968. [ Google Scholar ] [ CrossRef ] [ Green Version ]
- Downing, J.A.; Prairie, Y.T.; Cole, J.J.; Duarte, C.M.; Tranvik, L.J.; Striegl, R.G.; McDowell, W.H.; Kortelainen, P.; Caraco, N.F.; Melack, J.M.; et al. The global abundance and size distribution of lakes, ponds, and impoundments. Limnol. Oceanogr. 2006 , 51 , 2388–2397. [ Google Scholar ] [ CrossRef ] [ Green Version ]
- Robbins, S.J.; Hynek, B.M. A new global database of Mars impact craters ≥ 1 km: Database creation, properties, and parameters. J. Geophys. Res. Planets 2012 , 117 , E5. [ Google Scholar ] [ CrossRef ]
- Golombek, M.P.; Warner, N.H.; Ganti, V.; Lamb, M.P.; Parker, T.J.; Fergason, R.L.; Sullivan, R. Small crater modification on Meridiani Planum and implications for erosion rates and climate change on Mars. J. Geophys. Res. Planets 2014 , 119 , 2522–2547. [ Google Scholar ] [ CrossRef ]
- Fairén, A.G.; Dohm, J.M.; Baker, V.R.; Pablo, M.A.D.; Ruiz, J.; Ferris, J.C.; Anderson, R.C. Episodic flood inundations of the northern plains of Mars. Icarus 2003 , 165 , 53–67. [ Google Scholar ] [ CrossRef ] [ Green Version ]
- Pearce, B.K.; Tupper, A.S.; Pudritz, R.E.; Higgs, P.G. Constraining the time interval for the origin of life on Earth. Astrobiology 2018 , 18 , 343–364. [ Google Scholar ] [ CrossRef ] [ PubMed ]
- Fassett, C.I.; Head, I.I.I.J.W. Valley network-fed, open-basin lakes on Mars: Distribution and implications for Noachian surface and subsurface hydrology. Icarus 2008 , 198 , 37–56. [ Google Scholar ] [ CrossRef ] [ Green Version ]
- Goudge, T.A.; Head, J.W.; Mustard, J.F.; Fassett, C.I. An analysis of open-basin lake deposits on Mars: Evidence for the nature of associated lacustrine deposits and post-lacustrine modification processes. Icarus 2012 , 219 , 211–229. [ Google Scholar ] [ CrossRef ]
- Bristow, T.F.; Rampe, E.B.; Achilles, C.N.; Blake, D.F.; Chipera, S.J.; Craig, P.; Crisp, J.A.; Marais, D.J.D.; Downs, R.T.; Gellert, R.; et al. Clay mineral diversity and abundance in sedimentary rocks of Gale crater, Mars. Sci. Adv. 2018 , 4 , eaar3330. [ Google Scholar ] [ CrossRef ] [ PubMed ] [ Green Version ]
- Ruff, S.W.; Niles, P.B.; Alfano, F.; Clarke, A.B. Evidence for a Noachian-aged ephemeral lake in Gusev crater, Mars. Geology 2014 , 42 , 359–362. [ Google Scholar ] [ CrossRef ] [ Green Version ]
- Frydenvang, J.; Mangold, N.; Wiens, R.C.; Fraeman, A.A.; Edgar, L.A.; Fedo, C.M.; L’Haridon, J.; Bedford, C.C.; Gupta, S.; Grotzinger, J.P.; et al. The chemostratigraphy of the Murray formation and role of diagenesis at Vera Rubin ridge in Gale crater, Mars, as observed by the ChemCam instrument. J. Geophys. Res. Planets 2020 , 125 , e2019JE006320. [ Google Scholar ] [ CrossRef ]
- Rapin, W.; Dromart, G.; Rubin, D.; Deit, L.L.; Mangold, N.; Edgar, L.A.; Gasnault, O.; Herkenhoff, K.; Mouélic, S.L.; Anderson, R.B.; et al. Alternating wet and dry depositional environments recorded in the stratigraphy of Mount Sharp at Gale crater, Mars. Geology 2021 . [ Google Scholar ] [ CrossRef ]
- Martin, P.E.; Farley, K.A.; Malespin, C.A.; Mahaffy, P.R.; Edgett, K.S.; Gupta, S.; Dietrich, W.E.; Malin, M.C.; Stack, K.M.; Vasconcelos, P.M. Billion-year exposure ages in Gale crater (Mars) indicate Mount Sharp formed before the Amazonian period. Earth Planet. Sci. Lett. 2021 , 554 , 116667. [ Google Scholar ] [ CrossRef ]
- Milliken, R.E.; Grotzinger, J.P.; Wiens, R.; Gellert, R.; Thompson, L.M.; Sheppard, R.; Vasavada, A.; Bristow, T.; Mangold, N. The chemistry and mineralogy of an ancient lacustrine sequence on Mars: Lessons learned from integrating rover and orbiter datasets. LPI Contrib. 2019 , 2089 , 6191. [ Google Scholar ]
- Hurowitz, J.A.; Grotzinger, J.P.; Fischer, W.W.; McLennan, S.M.; Milliken, R.E.; Stein, N.; Vasavada, A.R.; Blake, D.F.; Dehouck, E.; Eigenbrode, J.L.; et al. Redox stratification of an ancient lake in Gale crater, Mars. Science 2017 , 356 , 6341. [ Google Scholar ] [ CrossRef ] [ Green Version ]
- Alfreider, A.; Baumer, A.; Bogensperger, T.; Posch, T.; Salcher, M.M.; Summerer, M. CO 2 assimilation strategies in stratified lakes: Diversity and distribution patterns of chemolithoautotrophs. Environ. Microbiol. 2017 , 19 , 2754–2768. [ Google Scholar ] [ CrossRef ] [ Green Version ]
- Stein, N.; Grotzinger, J.P.; Schieber, J.; Mangold, N.; Hallet, B.; Newsom, H.; Stack, K.M.; Berger, J.A.; Thompson, L.; Siebach, K.L.; et al. Desiccation cracks provide evidence of lake drying on Mars, Sutton Island member, Murray formation, Gale Crater. Geology 2018 , 46 , 515–518. [ Google Scholar ] [ CrossRef ]
- Martin, P.E.; Farley, K.A.; Archer, D.P., Jr.; Hogancamp, J.V.; Siebach, K.L.; Grotzinger, J.P.; McLennan, S.M. Reevaluation of perchlorate in Gale crater rocks suggests geologically recent perchlorate addition. J. Geophys. Res. Planets 2020 , 125 , e2019JE006156. [ Google Scholar ] [ CrossRef ]
- Greeley, R.; Schneid, B.D. Magma generation on Mars: Amounts, rates, and comparisons with Earth, Moon, and Venus. Science 1991 , 254 , 996–998. [ Google Scholar ] [ CrossRef ]
- Newsom, H.E. Hydrothermal alteration of impact melt sheets with implications for Mars. Icarus 1980 , 44 , 207–216. [ Google Scholar ] [ CrossRef ]
- Ruff, S.W.; Campbell, K.A.; Van Kranendonk, M.J.; Rice, M.S.; Farmer, J.D. The case for ancient hot springs in Gusev crater, Mars. Astrobiology 2020 , 20 , 475–499. [ Google Scholar ] [ CrossRef ] [ PubMed ] [ Green Version ]
- Rapin, W.; Chauviré, B.; Gabriel, T.S.; McAdam, A.C.; Ehlmann, B.L.; Hardgrove, C.; Meslin, P.Y.; Rondeau, B.; Dehouck, E.; Franz, H.B.; et al. In situ analysis of opal in Gale Crater, Mars. J. Geophys. Res. Planets 2018 , 123 , 1955–1972. [ Google Scholar ] [ CrossRef ]
- Sun, V.Z.; Milliken, R.E. Characterizing the mineral assemblages of hot spring environments and applications to Mars orbital data. Astrobiology 2020 , 20 , 453–474. [ Google Scholar ] [ CrossRef ]
- Rossi, A.P.; Neukum, G.; Pondrelli, M.; Van Gasselt, S.; Zegers, T.; Hauber, E.; Chicarro, A.; Foing, B. Large-scale spring deposits on Mars? J. Geophys. Res. Planets 2008 , 113 , E8. [ Google Scholar ] [ CrossRef ]
- Berger, J.A.; Schmidt, M.E.; Gellert, R.; Boyd, N.I.; Desouza, E.D.; Flemming, R.L.; Izawa, M.R.; Ming, D.W.; Perrett, G.M.; Rampe, E.B.; et al. Zinc and germanium in the sedimentary rocks of Gale Crater on Mars indicate hydrothermal enrichment followed by diagenetic fractionation. J. Geophys. Res. Planets 2017 , 122 , 1747–1772. [ Google Scholar ] [ CrossRef ]
- Michalski, J.R.; Onstott, T.C.; Mojzsis, S.J.; Mustard, J.; Chan, Q.H.; Niles, P.B.; Johnson, S.S. The Martian subsurface as a potential window into the origin of life. Nat. Geosci. 2018 , 11 , 21–26. [ Google Scholar ] [ CrossRef ]
- Bridges, J.C.; Schwenzer, S.P. The nakhlite hydrothermal brine on Mars. Earth Planet. Sci. Lett. 2012 , 359 , 117–123. [ Google Scholar ] [ CrossRef ]
- Amador, E.S.; Bandfield, J.L.; Brazelton, W.J.; Kelley, D. The lost city hydrothermal field: A spectroscopic and astrobiological analogue for Nili Fossae, Mars. Astrobiology 2017 , 17 , 1138–1160. [ Google Scholar ] [ CrossRef ]
- Brown, A.J.; Hook, S.J.; Baldridge, A.M.; Crowley, J.K.; Bridges, N.T.; Thomson, B.J.; Marion, G.M.; Filho, C.R.D.S.; Bishop, J.L. Hydrothermal formation of clay-carbonate alteration assemblages in the Nili Fossae region of Mars. Earth Planet. Sci. Lett. 2010 , 297 , 174–182. [ Google Scholar ] [ CrossRef ] [ Green Version ]
- Turner, S.M.; Bridges, J.C.; Grebby, S.; Ehlmann, B.L. Hydrothermal activity recorded in post Noachian-aged impact craters on Mars. J. Geophys. Res. Planets 2016 , 121 , 608–625. [ Google Scholar ] [ CrossRef ] [ Green Version ]
- Carrozzo, F.G.; Achille, G.D.; Salese, F.; Altieri, F.; Bellucci, G. Geology and mineralogy of the Auki Crater, Tyrrhena Terra, Mars: A possible post impact-induced hydrothermal system. Icarus 2017 , 281 , 228–239. [ Google Scholar ] [ CrossRef ]
- Hamilton, C.W.; Mouginis-Mark, P.J.; Sori, M.M.; Scheidt, S.P.; Bramson, A.M. Episodes of aqueous flooding and effusive volcanism associated with Hrad Vallis, Mars. J. Geophys. Res. Planets 2018 , 123 , 1484–1510. [ Google Scholar ] [ CrossRef ] [ Green Version ]
- Michalski, J.R.; Dobrea, E.Z.; Niles, P.B.; Cuadros, J. Ancient hydrothermal seafloor deposits in Eridania basin on Mars. Nat. Commun. 2017 , 8 , 1–10. [ Google Scholar ] [ CrossRef ] [ PubMed ] [ Green Version ]
- Azuma, S.; Katayama, I. Evolution of the rheological structure of Mars. Earth Planets Space 2017 , 69 , 1–3. [ Google Scholar ] [ CrossRef ] [ Green Version ]
- Quarles, B.L.; Lissauer, J.J. Dynamical evolution of the Earth–Moon progenitors–Whence Theia? Icarus 2015 , 248 , 318–339. [ Google Scholar ] [ CrossRef ] [ Green Version ]
- Morrison, P.R.; Mojzsis, S.J. Tracing the early emergence of microbial sulfur metabolisms. Geomicrobiol. J. 2020 , 10 , 1–21. [ Google Scholar ] [ CrossRef ]
- Bouvier, L.C.; Costa, M.M.; Connelly, J.N.; Jensen, N.K.; Wielandt, D.; Storey, M.; Nemchin, A.A.; Whitehouse, M.J.; Snape, J.F.; Bellucci, J.J.; et al. Evidence for extremely rapid magma ocean crystallization and crust formation on Mars. Nature 2018 , 558 , 586–589. [ Google Scholar ] [ CrossRef ] [ PubMed ] [ Green Version ]
- Quantin-Nataf, C.; Craddock, R.A.; Dubuffet, F.; Lozac’h, L.; Martinot, M. Decline of crater obliteration rates during early martian history. Icarus 2019 , 317 , 427–433. [ Google Scholar ] [ CrossRef ]
- Grant, J.A.; Wilson, S.A. Evidence for late alluvial activity in Gale crater, Mars. Geophys. Res. Lett. 2019 , 46 , 7287–7294. [ Google Scholar ] [ CrossRef ]
- Martin, P.E.; Farley, K.A.; Baker, M.B.; Malespin, C.A.; Schwenzer, S.P.; Cohen, B.A.; Mahaffy, P.R.; McAdam, A.C.; Ming, D.W.; Vasconcelos, P.M.; et al. A two-step K-Ar experiment on Mars: Dating the diagenetic formation of jarosite from Amazonian groundwaters. J. Geophys. Res. Planets 2017 , 122 , 2803–2818. [ Google Scholar ] [ CrossRef ]
- Guitreau, M.; Flahaut, J. Record of low-temperature aqueous alteration of Martian zircon during the late Amazonian. Nat. Commun. 2019 , 10 , 1–9. [ Google Scholar ] [ CrossRef ]
- Richardson, M.I.; Mischna, M.A. Long-term evolution of transient liquid water on Mars. J. Geophys. Res. Planets 2005 , 110 , E3. [ Google Scholar ] [ CrossRef ] [ Green Version ]
- Davila, A.; Kahre, M.A.; Quinn, R.; Marais, D.J.D. The biological potential of present-day Mars. Planetary Astrobiology 2020 , 16 , 169. [ Google Scholar ]
- Meadows, V.; Arney, G.; Schmidt, B.; Marais, D.J.D. (Eds.) Planetary Astrobiology ; University of Arizona Press: San Diego, CA, USA, 2020. [ Google Scholar ]
- Malin, M.C.; Edgett, K.S.; Posiolova, L.V.; McColley, S.M.; Dobrea, E.Z. Present-day impact cratering rate and contemporary gully activity on Mars. Science 2006 , 314 , 1573–1577. [ Google Scholar ] [ CrossRef ] [ PubMed ]
- Dundas, C.M.; Byrne, S.; McEwen, A.S.; Mellon, M.T.; Kennedy, M.R.; Daubar, I.J.; Saper, L. HiRISE observations of new impact craters exposing Martian ground ice. J. Geophys. Res. Planets 2014 , 119 , 109–127. [ Google Scholar ] [ CrossRef ]
- Vaughan, R.G.; Heasler, H.; Jaworowski, C.; Lowenstern, J.B.; Keszthelyi, L.P. Provisional Map of Thermal Areas in Yellowstone National Park Based on Satellite Thermal Infrared Imaging and Field Observations ; US Geological Survey Scientific Investigations Report, No. 5137; US Geological Survey: Washington, DC, USA, 2014; p. 2. [ Google Scholar ] [ CrossRef ]
- Benner, S.A.; Devine, K.G.; Matveeva, L.N.; Powell, D.H. The missing organic molecules on Mars. Proc. Natl. Acad. Sci. USA 2000 , 97 , 2425–2430. [ Google Scholar ] [ CrossRef ] [ PubMed ] [ Green Version ]
- Zent, A. A historical search for habitable ice at the Phoenix landing site. Icarus 2008 , 196 , 385–408. [ Google Scholar ] [ CrossRef ]
- Krasnopolsky, V.A.; Feldman, P.D. Detection of molecular hydrogen in the atmosphere of Mars. Science 2001 , 294 , 1914–1917. [ Google Scholar ] [ CrossRef ] [ PubMed ]
- Grew, E.S.; Bada, J.L.; Hazen, R.M. Borate minerals and origin of the RNA world. Origins Life Evol. Biosph. 2011 , 41 , 307–316. [ Google Scholar ] [ CrossRef ] [ PubMed ]
- Horneck, G.; Bäcker, H. Can microorganisms withstand the multistep trial of interplanetary transfer? Considerations and experimental approaches. Origins Life Evol. Biosph. 1986 , 16 , 414–415. [ Google Scholar ] [ CrossRef ]
- Clark, B.C. Barriers to natural interchange of biologically active material between Earth and Mars. Origins Life 1986 , 16 , 410–411. [ Google Scholar ] [ CrossRef ]
- Mileikowsky, C.; Cucinotta, F.A.; Wilson, J.W.; Gladman, B.; Horneck, G.; Lindegren, L.; Melosh, J.; Rickman, H.; Valtonen, M.; Zheng, J.Q. Natural transfer of viable microbes in space: From Mars to Earth and Earth to Mars. Icarus 2000 , 145 , 391–427. [ Google Scholar ] [ CrossRef ]
- Clark, B.C. Planetary interchange of bioactive material: Probability factors and implications. Origins Life Evol. Biosph. 2001 , 31 , 185–197. [ Google Scholar ] [ CrossRef ] [ PubMed ]
- Kirschvink, J.L.; Weiss, B.P. Mars, panspermia, and the origin of life: Where did it all begin. Palaeontol. Electron. 2002 , 4 , 8–15. [ Google Scholar ]
- Melosh, H.J. The rocky road to panspermia. Nature 1988 , 332 , 687–688. [ Google Scholar ] [ CrossRef ] [ PubMed ]
- Gladman, B.J.; Burns, J.A.; Duncan, M.; Lee, P.; Levison, H.F. The exchange of impact ejecta between terrestrial planets. Science 1996 , 271 , 1387–1392. [ Google Scholar ] [ CrossRef ]
- El Goresy, A.; Gillet, P.; Miyahara, M.; Ohtani, E.; Ozawa, S.; Beck, P.; Montagnac, G. Shock-induced deformation of Shergottites: Shock-pressures and perturbations of magmatic ages on Mars. Geochim. Cosmochim. Acta 2013 , 101 , 233–262. [ Google Scholar ] [ CrossRef ]
- Cabrol, N.A.; Grin, E.A. (Eds.) From Habitability to Life on Mars ; Elsevier: Amsterdam, The Netherlands, 2018. [ Google Scholar ]
- Westall, F.; Foucher, F.; Bost, N.; Bertrand, M.; Loizeau, D.; Vago, J.L.; Kminek, G.; Gaboyer, F.; Campbell, K.A.; Bréhéret, J.G.; et al. Biosignatures on Mars: What, where, and how? Implications for the search for martian life. Astrobiology 2015 , 15 , 998–1029. [ Google Scholar ] [ CrossRef ]
Click here to enlarge figure
MDPI stays neutral with regard to jurisdictional claims in published maps and institutional affiliations. |
Share and Cite
Clark, B.C.; Kolb, V.M.; Steele, A.; House, C.H.; Lanza, N.L.; Gasda, P.J.; VanBommel, S.J.; Newsom, H.E.; Martínez-Frías, J. Origin of Life on Mars: Suitability and Opportunities. Life 2021 , 11 , 539. https://doi.org/10.3390/life11060539
Clark BC, Kolb VM, Steele A, House CH, Lanza NL, Gasda PJ, VanBommel SJ, Newsom HE, Martínez-Frías J. Origin of Life on Mars: Suitability and Opportunities. Life . 2021; 11(6):539. https://doi.org/10.3390/life11060539
Clark, Benton C., Vera M. Kolb, Andrew Steele, Christopher H. House, Nina L. Lanza, Patrick J. Gasda, Scott J. VanBommel, Horton E. Newsom, and Jesús Martínez-Frías. 2021. "Origin of Life on Mars: Suitability and Opportunities" Life 11, no. 6: 539. https://doi.org/10.3390/life11060539
Article Metrics
Article access statistics, further information, mdpi initiatives, follow mdpi.
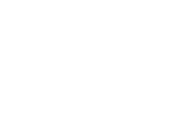
Subscribe to receive issue release notifications and newsletters from MDPI journals

Signs of Life on Mars? NASA’s Perseverance Rover Begins the Hunt
Your browser cannot play the provided video file(s).
The robotic arm on NASA’s Perseverance rover reached out to examine rocks in an area on Mars nicknamed the “Cratered Floor Fractured Rough” area in this image captured on July 10, 2021 (the 138th sol, or Martian day, of its mission).
After testing a bristling array of instruments on its robotic arm, NASA’s latest Mars rover gets down to business: probing rocks and dust for evidence of past life.
NASA’s Mars 2020 Perseverance rover has begun its search for signs of ancient life on the Red Planet. Flexing its 7-foot (2-meter) mechanical arm, the rover is testing the sensitive detectors it carries, capturing their first science readings. Along with analyzing rocks using X-rays and ultraviolet light, the six-wheeled scientist will zoom in for closeups of tiny segments of rock surfaces that might show evidence of past microbial activity.
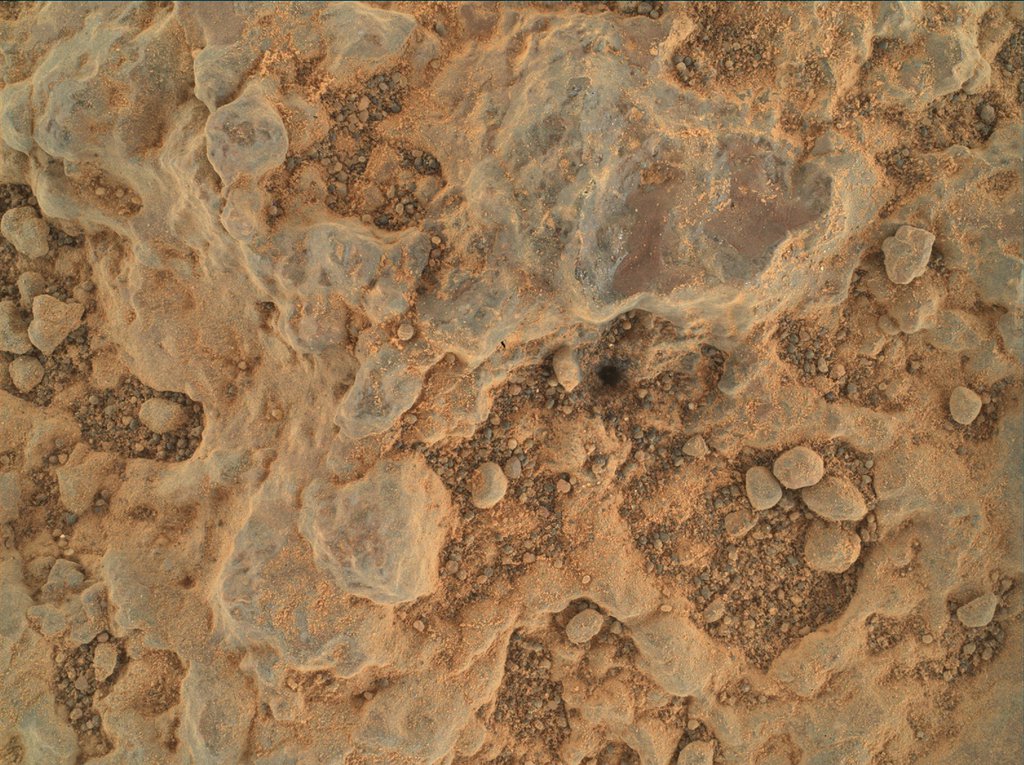
NASA’s Perseverance Mars rover took this close-up of a rock target nicknamed “Foux” using its WATSON camera on the end of the rover’s robotic arm. The image was taken July 11, 2021, the 139th Martian day, or sol, of the mission.
Called PIXL , or Planetary Instrument for X-ray Lithochemistry, the rover’s X-ray instrument delivered unexpectedly strong science results while it was still being tested, said Abigail Allwood, PIXL’s principal investigator at NASA’s Jet Propulsion Laboratory in Southern California. Located at the end of the arm, the lunchbox-size instrument fired its X-rays at a small calibration target – used to test instrument settings – aboard Perseverance and was able to determine the composition of Martian dust clinging to the target.
“We got our best-ever composition analysis of Martian dust before it even looked at rock,” Allwood said.
That’s just a small taste of what PIXL, combined with the arm’s other instruments, is expected to reveal as it zeroes in on promising geological features over the weeks and months ahead.
Scientists say Jezero Crater was a crater lake billions of years ago, making it a choice landing site for Perseverance. The crater has long since dried out, and the rover is now picking its way across its red, broken floor .
“If life was there in Jezero Crater, the evidence of that life could be there,” said Allwood, a key member of the Perseverance “arm science” team.
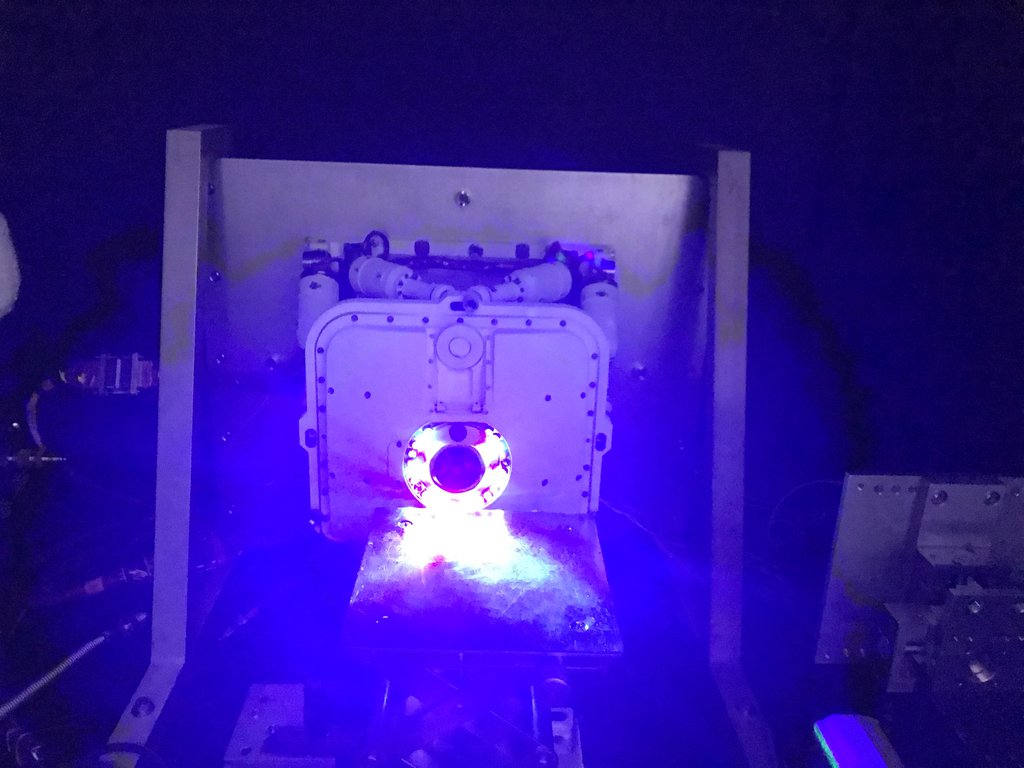
PIXL, one of seven instruments aboard NASA's Perseverance Mars rover, is equipped with light diodes circling its opening to take pictures of rock targets in the dark. Using artificial intelligence, PIXL relies on the images to determine how far away it is from a target to be scanned.
To get a detailed profile of rock textures, contours, and composition, PIXL’s maps of the chemicals throughout a rock can be combined with mineral maps produced by the SHERLOC instrument and its partner, WATSON. SHERLOC – short for Scanning Habitable Environments with Raman & Luminescence for Organics & Chemicals – uses an ultraviolet laser to identify some of the minerals in the rock, while WATSON takes closeup images that scientists can use to determine grain size, roundness, and texture, all of which can help determine how the rock was formed.
Early WATSON closeups have already yielded a trove of data from Martian rocks, the scientists said, such as a variety of colors, sizes of grains in the sediment, and even the presence of “cement” between the grains. Such details can provide important clues about formation history, water flow, and ancient, potentially habitable Martian environments. And combined with those from PIXL, they can provide a broader environmental and even historical snapshot of Jezero Crater.
“What is the crater floor made out of? What were the conditions like on the crater floor?” asks Luther Beegle of JPL, SHERLOC’s principal investigator. “That does tell us a lot about the early days of Mars, and potentially how Mars formed. If we have an idea of what the history of Mars is like, we’ll be able to understand the potential for finding evidence of life.”
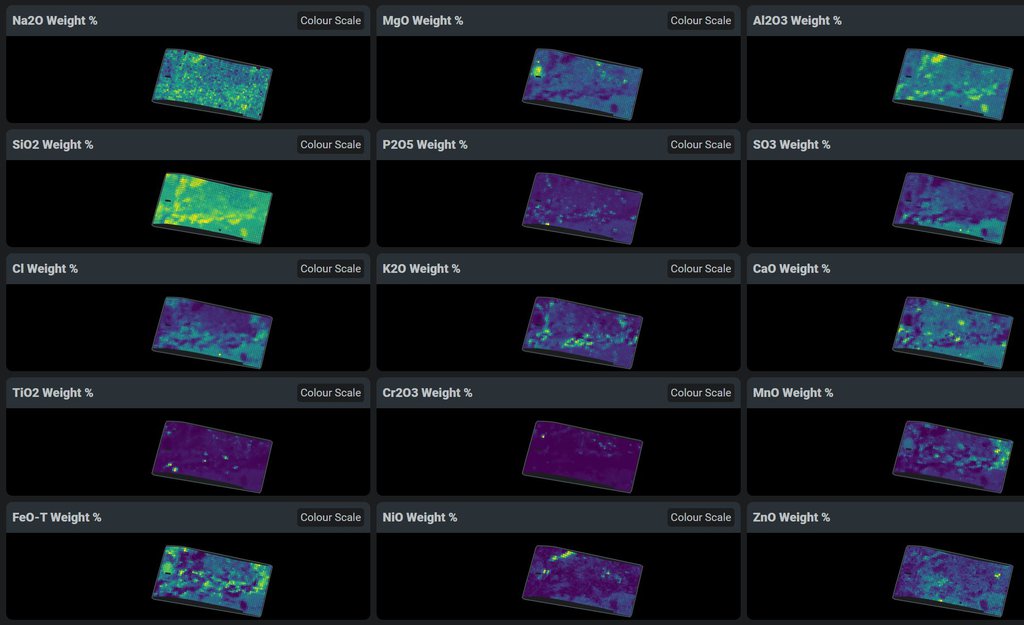
This data shows chemicals detected within a single rock on Mars by PIXL, one of the instruments on the end of the robotic arm aboard NASA’s Perseverance Mars rover. PIXL allows scientists to study where specific chemicals can be found within an area as small as a postage stamp.
The Science Team
While the rover has significant autonomous capabilities, such as driving itself across the Martian landscape, hundreds of earthbound scientists are still involved in analyzing results and planning further investigations.
“There are almost 500 people on the science team,” Beegle said. “The number of participants in any given action by the rover is on the order of 100. It’s great to see these scientists come to agreement in analyzing the clues, prioritizing each step, and putting together the pieces of the Jezero science puzzle.”
That will be critical when the Mars 2020 Perseverance rover collects its first samples for eventual return to Earth. They’ll be sealed in superclean metallic tubes on the Martian surface so that a future mission could collect them and send back to the home planet for further analysis.
Despite decades of investigation on the question of potential life, the Red Planet has stubbornly kept its secrets.
“Mars 2020, in my view, is the best opportunity we will have in our lifetime to address that question,” said Kenneth Williford, the deputy project scientist for Perseverance.
The geological details are critical, Allwood said, to place any indication of possible life in context, and to check scientists’ ideas about how a second example of life’s origin could come about.
Combined with other instruments on the rover, the detectors on the arm, including SHERLOC and WATSON, could make humanity’s first discovery of life beyond Earth.
More About the Mission
A key objective for Perseverance’s mission on Mars is astrobiology , including the search for signs of ancient microbial life. The rover will characterize the planet’s geology and past climate, pave the way for human exploration of the Red Planet, and be the first mission to collect and cache Martian rock and regolith (broken rock and dust).
Subsequent NASA missions, in cooperation with ESA (European Space Agency), would send spacecraft to Mars to collect these sealed samples from the surface and return them to Earth for in-depth analysis.
The Mars 2020 Perseverance mission is part of NASA’s Moon to Mars exploration approach, which includes Artemis missions to the Moon that will help prepare for human exploration of the Red Planet.
JPL, which is managed for NASA by Caltech in Pasadena, California, built and manages operations of the Perseverance rover.
For more about Perseverance:
mars.nasa.gov/mars2020/
nasa.gov/perseverance
News Media Contact
Jet Propulsion Laboratory, Pasadena, Calif.
818-393-9011
April 5, 2023
NASA’s Perseverance Rover May Already Have Evidence of Ancient Martian Life
A half-kilogram’s worth of samples gathered by NASA’s Perseverance rover for eventual return to Earth holds weighty implications for life on Mars
By Jonathan O'Callaghan
NASA’s Perseverance Mars rover took a selfie with several of the 10 sample tubes it deposited at a sample depot it is creating within an area of Jezero Crater nicknamed Three Forks.
NASA/JPL-Caltech/MSSS
If life ever existed on Mars, we may already have the answer at hand. In January NASA’s Perseverance rover deposited 10 tubes on the surface of Mars. Each contains a sample of Martian rock that was carefully selected for its potential to clarify chapters of the planet’s still-murky history. Those tubes “are capable of telling us whether Mars was habitable,” says Mitch Schulte, Perseverance’s program scientist at NASA Headquarters in Washington, D.C. “We see evidence of particular minerals that tell us there was water. Some of these minerals indicate there was organic material.”
But to know for sure, scientists need to bring these tubes back to Earth for closer study—an audacious endeavor known as Mars Sample Return (MSR), which is slated for the early 2030s via a follow-up robotic mission . These 10 tubes are only the opening course in a bigger awaiting feast, a backup cache in case Perseverance breaks down before it can fill and deliver the 33 additional tubes that it carries. These tubes will hold samples sourced from the area in and around Jezero Crater, the site of a four-billion-year-old river delta and the locale where the rover landed on February 18, 2021. Although many of the samples are yet to be gathered for a journey to Earth that remains years in the future, those already collected have whetted researchers’ appetite for their return home.
Scientists targeted Jezero for Perseverance because, on our planet, sprawling river systems like that found in the Martian crater build up enormous deposits of sediments. Washed in from a sizable swath of the surrounding landscape, these deposits contain various minerals that can be used to chart the Red Planet’s past geology. Also most anywhere water is found on Earth, life accompanies it. The same might hold true for Mars, meaning Jezero’s sediments could conceivably harbor biological remains. “We’re looking for signs of habitability—liquid water and the raw materials of life,” says Mark Sephton of Imperial College London, a member of the rover’s sampling team.
On supporting science journalism
If you're enjoying this article, consider supporting our award-winning journalism by subscribing . By purchasing a subscription you are helping to ensure the future of impactful stories about the discoveries and ideas shaping our world today.
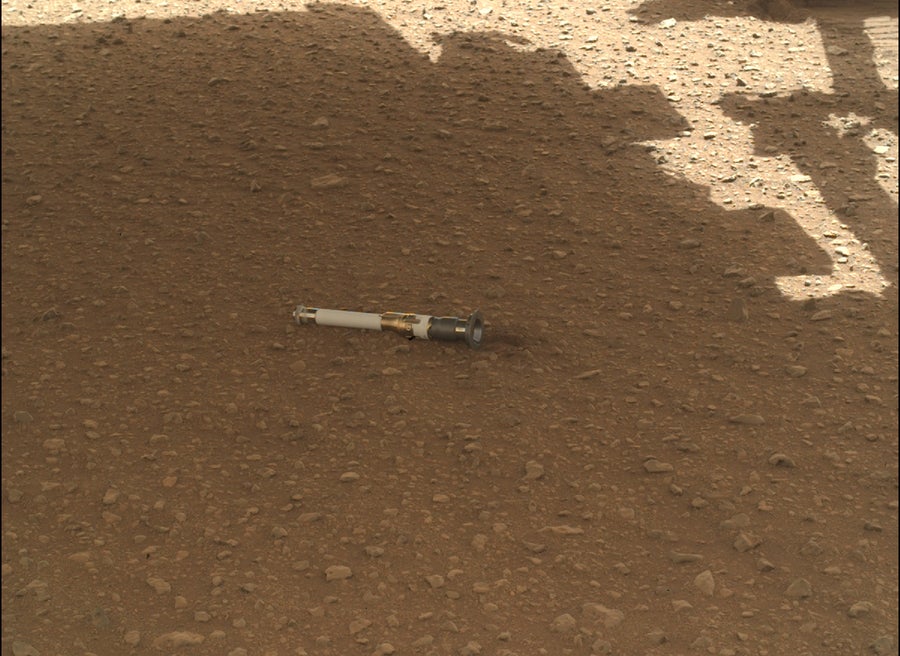
A close-up of one of the Perseverance Mars rover’s 43 sample tubes, deposited for future retrieval on the surface of Mars. Credit: NASA/JPL-Caltech/MSSS
Perseverance collects most of its samples using a small drill, producing chalk-stick-sized specimens that each fit within cigarlike tubes measuring less than 15 centimeters long. Of the 43 sample tubes, 38 are slated for samples from the surface, with the remaining five being “witness tubes” to catch whiffs of Martian air and check for any contaminating gases that might vent from the rover. Collected in September 2021 , the rover’s first sample is thought to be igneous rock from an ancient lava flow. Studying this material should allow scientists to date the crater more precisely. Since then, the rover has filled nearly half of its remaining tubes as it journeys several kilometers further up the ancient river’s channels toward Jezero Crater’s rim.
As a contingency, 10 of the samples are duplicates, each paired with another sample taken from the same location. These are the tubes Perseverance dropped on the surface as backup for potential future retrieval. In December 2022, in one of the last decisions he’d make at the space agency before reentering the private sector, NASA’s then science chief Thomas Zurbuchen made the call to drop that cache at a location called Three Forks. The surface drop-off was completed at the end of January, around the same time Perseverance officially began the “extended” phase of its mission—and after the science team agreed that those 10 samples alone could answer the question of past habitability if needed. MSR’s optimal plan calls for the rover to carry its remaining tubes to a yet-to-be-built lander slated to touch down in Jezero’s vicinity around 2030. Once the lander has secured those samples, it will launch them on a rocket back to Earth .
“We want the ones on the rover to come back,” Zurbuchen says. “But even the ones on the surface check all the boxes.” That includes igneous rock to date the crater and sedimentary rock and clays that may contain biosignatures, perhaps even fossilized evidence of microbial life. “They’re already worth the $10-billion investment,” Zurbuchen says, citing the MSR program’s estimated total cost. Some of the most promising samples are from a location called Wildcat Ridge, a meter-wide rock that contains evidence of sulfates. “Those are the ones we’re most excited about in terms of potential biosignatures,” says Kathleen Benison of West Virginia University, who is part of Perseverance’s sampling team. “Sulfate minerals can grow from groundwater. On Earth, those kinds of waters tend to have a lot of microbial life,” which can be entombed and preserved in sulfate minerals.
Besides sulfates, life-seeking scientists are particularly eager to grab samples from mudstones—fine-grained sedimentary rocks that the Curiosity rover has seen in Gale Crater but that Perseverance has not yet spotted. “Microbe cells are tiny,” says Tanja Bosak of the Massachusetts Institute of Technology, who is also part of the sampling team. “The mineral grain size should be even finer to preserve the [fossil] shape instead of destroying it. If you roll a boulder over a person, you will smush that person into something unrecognizable. For a microbe, everything is a boulder—unless you’re talking about mudstones.” The team members are also keen to sample carbonates, similar to things like chalk and limestone on Earth, which could preserve biosignatures as well. “If there had been microbial life in the lake, [the carbonates] could have trapped microbial matter in it,” says Sanjeev Gupta of Imperial College London, who is one of the “long-term planners” who plot out the rover’s path. On March 30, Perseverance collected its first carbonate sample, from a rock named “Berea” thought to have formed from material washed into Jezero by the ancient river.
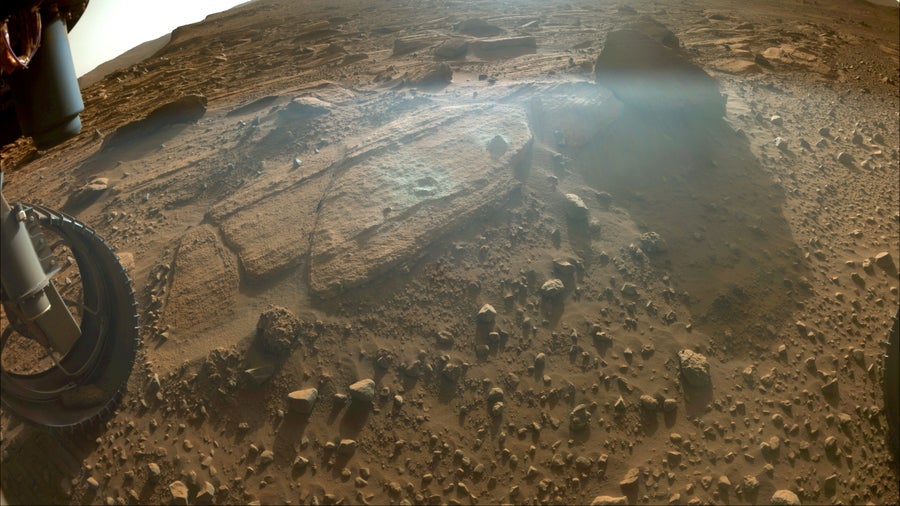
Taken on March 30, 2023, this image shows the rocky outcrop the Perseverance science team calls “Berea” after the NASA Mars rover extracted a carbonate rock core ( right ) and abraded a circular patch ( left ). Credit: NASA/JPL-Caltech
While Perseverance has been hard at work collecting samples on Mars, the return phase of the mission remains in flux. Originally, NASA had planned for a European-built “fetch” rover to land on Mars around 2030, collect the samples from Perseverance and return them to a capsule on the lander for launch. Once in orbit, the sample capsule would rendezvous with a European orbiter, which would ferry the samples back to Earth for a landing in 2033. These plans were complicated, however, by Russia’s invasion of Ukraine in 2022. In response to Russia’s aggression, European Space Agency (ESA) officials chose to step back from a partnership with the nation on another long-simmering Mars mission, the Rosalind Franklin ExoMars rover. Russia had been due to provide the rover’s nuclear power source, as well as the launch vehicle and landing platform. NASA has now agreed to supply such missing pieces and has sought funding to do so in its budgetary request to Congress last month. But this unanticipated assistance comes at the cost of the fetch rover. “We couldn’t do both,” Zurbuchen says. “We could not individually land the fetch rover and do ExoMars.”
The ExoMars mission, most everyone agrees, is eminently worth saving. The Rosalind Franklin rover will carry a drill that can augur two meters beneath the Martian surface, accessing a subterranean habitat for past and present life that is considerably less hostile than the surface. “Nobody has ever done that on Mars,” Zurbuchen says. “Our science community thinks it’s really important.”
Jorge Vago, ESA’s ExoMars project scientist in the Netherlands, was glad that NASA stepped in. To hit a target launch date of 2028, set forth by European member states in order to save the mission, “we need the American contributions,” Vago says. “It’s an amazing mission. If we find super interesting stuff that’s suggestive of a possible biological origin, I would expect we may want to have another sample return mission and bring back samples from the subsurface.”
NASA’s current MSR plan faces its own challenges. In a mid-March town hall hosted by NASA’s Science Mission Directorate, Jeff Gramling, MSR program director at NASA Headquarters, said that some aspects of the mission may need to be “descoped.” This would be a preventative measure to keep budgets under control. NASA’s annual request of nearly $1 billion for MSR is expected to grow in the next few years, raising fears that unchecked increases could force the space agency to siphon funds from unrelated missions. Descoping options include removing one of two “Marscopters” planned for MSR, which had been included to build on the wildly successful Ingenuity rotorcraft that is now approaching 50 flights on Mars . Among other tasks, MSR’s helicopters were added as a backup option for collecting the 10-tube sample cache at Three Forks. “The mission remains complex,” Gramling said during the town hall. “We’re working to our earliest possible launch date.”
Despite the overwhelmingly intricate logistics of seeking life on Mars, the scientific riches on offer have lost none of their luster. Perseverance’s returned samples will cumulatively be only about half a kilogram, but the weight of their implications is immeasurable. Will they reveal that a second genesis of life in the universe has unfolded on the surface of Mars? For that matter, will Rosalind Franklin, once it arrives, validate the long-held suspicion that Mars’s subsurface was—or still is—habitable, too? In our winding quest to determine if we are alone in the universe, the answer may be practically within our grasp, merely waiting for us to reach out to claim it. “We won’t know until we get the samples back,” Bosak says.
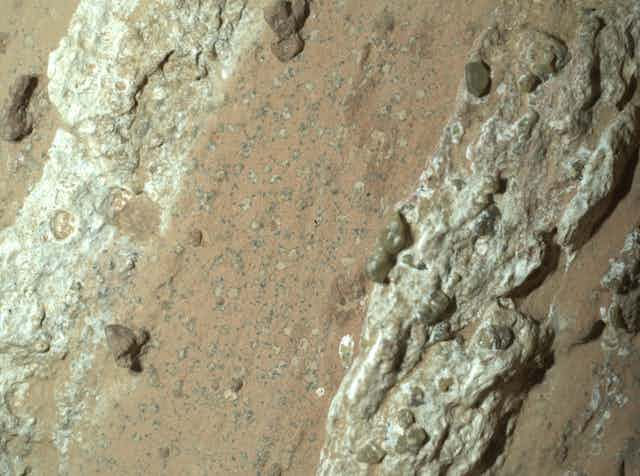
Has Nasa found evidence of ancient life on Mars? An expert examines the latest discovery
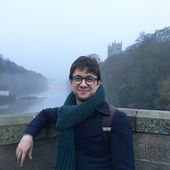
Reader in Astrobiology, The University of Edinburgh
Disclosure statement
Sean McMahon has received funding from Nasa.
The University of Edinburgh provides funding as a member of The Conversation UK.
View all partners
Nasa has announced the first detection of possible biosignatures in a rock on the surface of Mars. The rock contains the first martian organic matter to be decisively detected by the Perseverance rover , as well as curious discoloured spots that could indicate the past activity of microorganisms.
Ken Farley, project scientist on the mission, has called this “the most puzzling, complex, and potentially important rock yet investigated by Perseverance”.
Perseverance is part of Mars 2020, the first mission since Viking that is explicitly designed to seek life on Mars (officially, to “search for potential evidence of past life using observations regarding habitability and preservation as a guide”). Arguably, that objective has now been achieved: potential evidence for past life has been found. But much more work is needed to test this interpretation of the data. Here’s what we do know.
Since landing in Jezero crater a few years ago, Perseverance has traversed a series of rocks formed nearly four billion years before present. Mars back then was far more habitable than the cold, dry, toxic red planet of today.
There were thousands of rivers and lakes, a thick atmosphere, and comfortable temperatures and chemical conditions for life. Many of the rocks in Jezero are sedimentary: mud, silt and sand dumped by a river flowing into a lake.
The new discovery concerns one of these rocks. Informally named “Cheyava Falls” (a waterfall in Arizona), it is a small reddish block of what looks like a mudstone, enriched with organic molecules. The rock is also laced with parallel white veins. Between the veins are millimetre-scale whitish spots with dark rims. For an astrobiologist, all these features are intriguing. Let’s take them one-by-one.
First, “organic molecules” , are made of carbon and hydrogen (commonly with sulphur, oxygen or nitrogen as well). Examples include the proteins, fats, sugars, and nucleic acids from which all life as we know it is constructed.
Organic matter is common in rocks on Earth, most of it derived from the remains of ancient organisms. But the term “organic” is slightly misleading: such molecules can also be produced by non-biological reactions (in fact, we know this was happening four billion years ago on Mars).
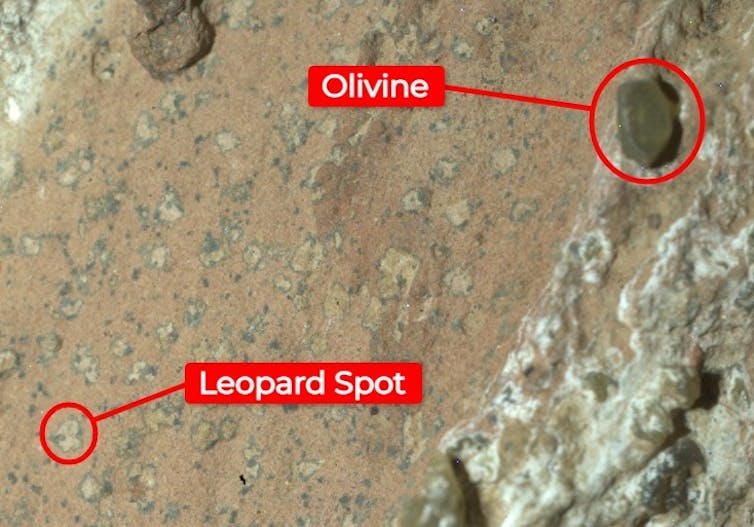
Simple non-biological organic molecules are common in the universe, and Nasa’s Curiosity rover already found them in mudstones in Gale Crater. They were also reportedly detected by Perseverance in Jezero crater last year.
Nevertheless, Ken Farley considers the new observation the first truly “compelling detection” of organics made by Perseverance. Nasa has not told us which types of organic molecules are actually present in Cheyava Falls, so it is hard to evaluate their origins. They could turn out to be biological, but a full analysis using laboratories on Earth would be needed to settle this question.
Next, the veins. These are composed of calcium sulphate, which precipitated like limescale when liquid water ran along fractures in the subsurface. Veins like these are common in Martian sedimentary rocks (Curiosity saw plenty of them), and of course they are not “biosignatures” even though they normally represent habitable conditions.
My own work has shown that microorganisms inhabiting subsurface fractures can produce chemical fossils that get trapped in calcium sulphate veins. Strangely, however, the veins in Cheyava Falls also contain olivine, an igneous mineral. This might suggest that the water was injected at temperatures too high for life. We need more data to know one way or the other.
Finally, what about those whitish, discoloured spots? These look like the “reduction spots”, also called “leopard spots”, commonly seen in red sedimentary rocks on Earth. Such rocks are rusty-red because they contain an oxidised form of iron. When chemical reactions modify the iron to a less oxidised state, it becomes soluble. Water carries the pigment away leaving a bleached spot behind.
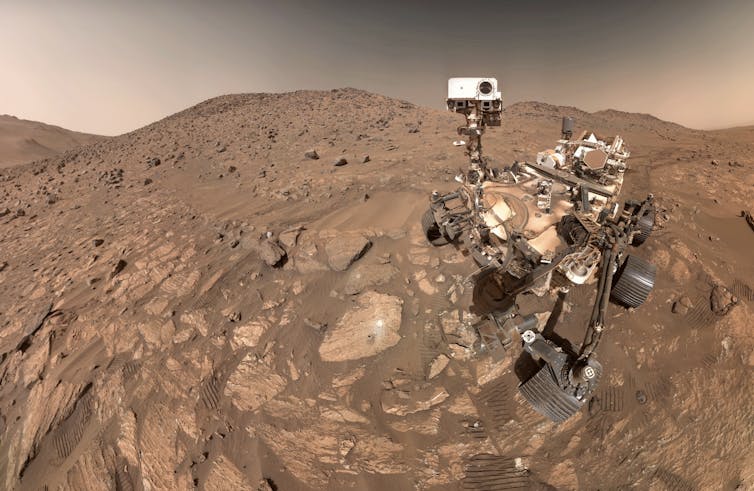
On Earth, these reactions are often driven by subsurface-dwelling bacteria. They use the oxidised iron as a source of energy, just as you and I use oxygen in the air. On Mars, bacteria-like organisms could have used the organic matter in the rock to complete the reaction (just as we use glucose from the food we eat).
Reduction spots haven’t been seen before on Mars, although bleached linear “halos” observed by Curiosity in Gale crater are somewhat similar. As one of the few astrobiologists to have studied reduction spots on Earth – and found evidence for biological processes within them – I am personally delighted. But as ever, caution is needed.
Potential non-biological causes need to be explored and ruled out. Iron-dissolving reactions can and do happen in sedimentary rocks without life. The dark margins of the Cheyava Falls spots are enriched in both iron and phosphate, an association previously suggested to occur around some calcium sulphate veins on Mars. This observation is consistent with life, but also with chemical reactions driven by acidic fluids.
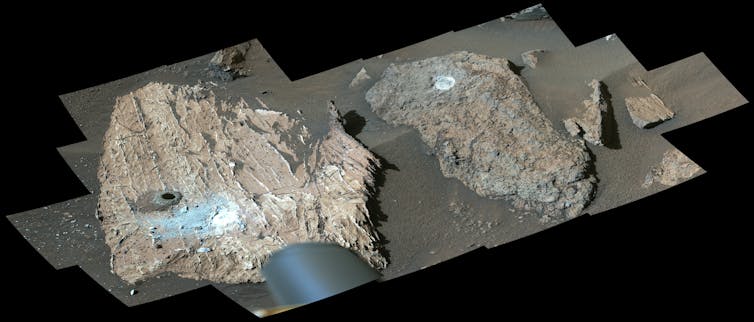
The new findings will nevertheless embolden those calling on Nasa and the European Space Agency to proceed with the troubled multi-billion-dollar sample retrieval programme , which Perseverance was supposed to begin. The rover has now cored out a piece of the Cheyava Falls rock. If current plans are realised – a big if – then future spacecraft will collect this piece (and others) and bring it to Earth.
It will then be analysed in state-of-the-art laboratories far more capable than the instruments aboard Perseverance. Until that happens, we cannot be sure whether Perseverance has really found fossils of ancient life on Mars. The evidence so far is not definitive, but it is certainly tantalising.
- Perseverance Rover
- Give me perspective

Community member - Training Delivery and Development Committee (Volunteer part-time)

Chief Executive Officer

Manager, Infrastructure Planning

Head of Evidence to Action

Supply Chain - Assistant/Associate Professor (Tenure-Track)
Essay on Life on Mars for Students and Children
500 words essay on life on mars.
Mars is the fourth planet from the sun in our solar system. Also, it is the second smallest planet in our solar system. The possibility of life on mars has aroused the interest of scientists for many years. A major reason for this interest is due to the similarity and proximity of the planet to Earth. Mars certainly gives some indications of the possibility of life.

Possibilities of Life on Mars
In the past, Mars used to look quite similar to Earth. Billions of years ago, there were certainly similarities between Mars and Earth. Furthermore, scientists believe that Mars once had a huge ocean. This ocean, experts believe, covered more of the planet’s surface than Earth’s own oceans do so currently.
Moreover, Mars was much warmer in the past that it is currently. Most noteworthy, warm temperature and water are two major requirements for life to exist. So, there is a high probability that previously there was life on Mars.
Life on Earth can exist in the harshest of circumstances. Furthermore, life exists in the most extreme places on Earth. Moreover, life on Earth is available in the extremely hot and dry deserts. Also, life exists in the extremely cold Antarctica continent. Most noteworthy, this resilience of life gives plenty of hope about life on Mars.
There are some ingredients for life that already exist on Mars. Bio signatures refer to current and past life markers. Furthermore, scientists are scouring the surface for them. Moreover, there has been an emergence of a few promising leads. One notable example is the presence of methane in Mars’s atmosphere. Most noteworthy, scientists have no idea where the methane is coming from. Therefore, a possibility arises that methane presence is due to microbes existing deep below the planet’s surface.
One important point to note is that no scratching of Mars’s surface has taken place. Furthermore, a couple of inches of scratching has taken place until now. Scientists have undertaken analysis of small pinches of soil. There may also have been a failure to detect signs of life due to the use of faulty techniques. Most noteworthy, there may be “refugee life” deep below the planet’s surface.
Get the huge list of more than 500 Essay Topics and Ideas
Challenges to Life on Mars
First of all, almost all plants and animals cannot survive the conditions on the surface of Mars. This is due to the extremely harsh conditions on the surface of Mars.
Another major problem is the gravity of Mars. Most noteworthy, the gravity on Mars is 38% to that of Earth. Furthermore, low gravity can cause health problems like muscle loss and bone demineralization.
The climate of Mars poses another significant problem. The temperature at Mars is much colder than Earth. Most noteworthy, the mean surface temperatures of Mars range between −87 and −5 °C. Also, the coldest temperature on Earth has been −89.2 °C in Antarctica.
Mars suffers from a great scarcity of water. Most noteworthy, water discovered on Mars is less than that on Earth’s driest desert.
Other problems include the high penetration of harmful solar radiation due to the lack of ozone layer. Furthermore, global dust storms are common throughout Mars. Also, the soil of Mars is toxic due to the high concentration of chlorine.
To sum it up, life on Mars is a topic that has generated a lot of curiosity among scientists and experts. Furthermore, establishing life on Mars involves a lot of challenges. However, the hope and ambition for this purpose are well alive and present. Most noteworthy, humanity must make serious efforts for establishing life on Mars.
FAQs on Life on Mars
Q1 State any one possibility of life on Mars?
A1 One possibility of life on Mars is the resilience of life. Most noteworthy, life exists in the most extreme places on Earth.
Q2 State anyone challenge to life on Mars?
A2 One challenge to life on Mars is a great scarcity of water.
Customize your course in 30 seconds
Which class are you in.

- Travelling Essay
- Picnic Essay
- Our Country Essay
- My Parents Essay
- Essay on Favourite Personality
- Essay on Memorable Day of My Life
- Essay on Knowledge is Power
- Essay on Gurpurab
- Essay on My Favourite Season
- Essay on Types of Sports
Leave a Reply Cancel reply
Your email address will not be published. Required fields are marked *
Download the App

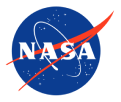
Suggested Searches
- Climate Change
- Expedition 64
- Mars perseverance
- SpaceX Crew-2
- International Space Station
- View All Topics A-Z
Humans in Space
Earth & climate, the solar system, the universe, aeronautics, learning resources, news & events.
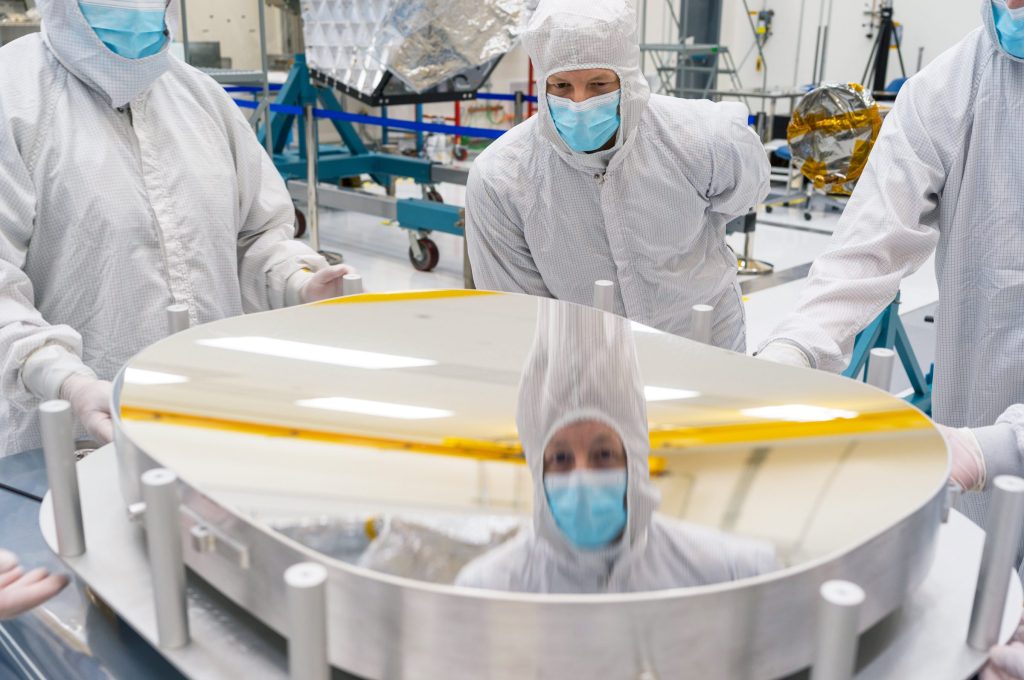
Work Is Under Way on NASA’s Next-Generation Asteroid Hunter
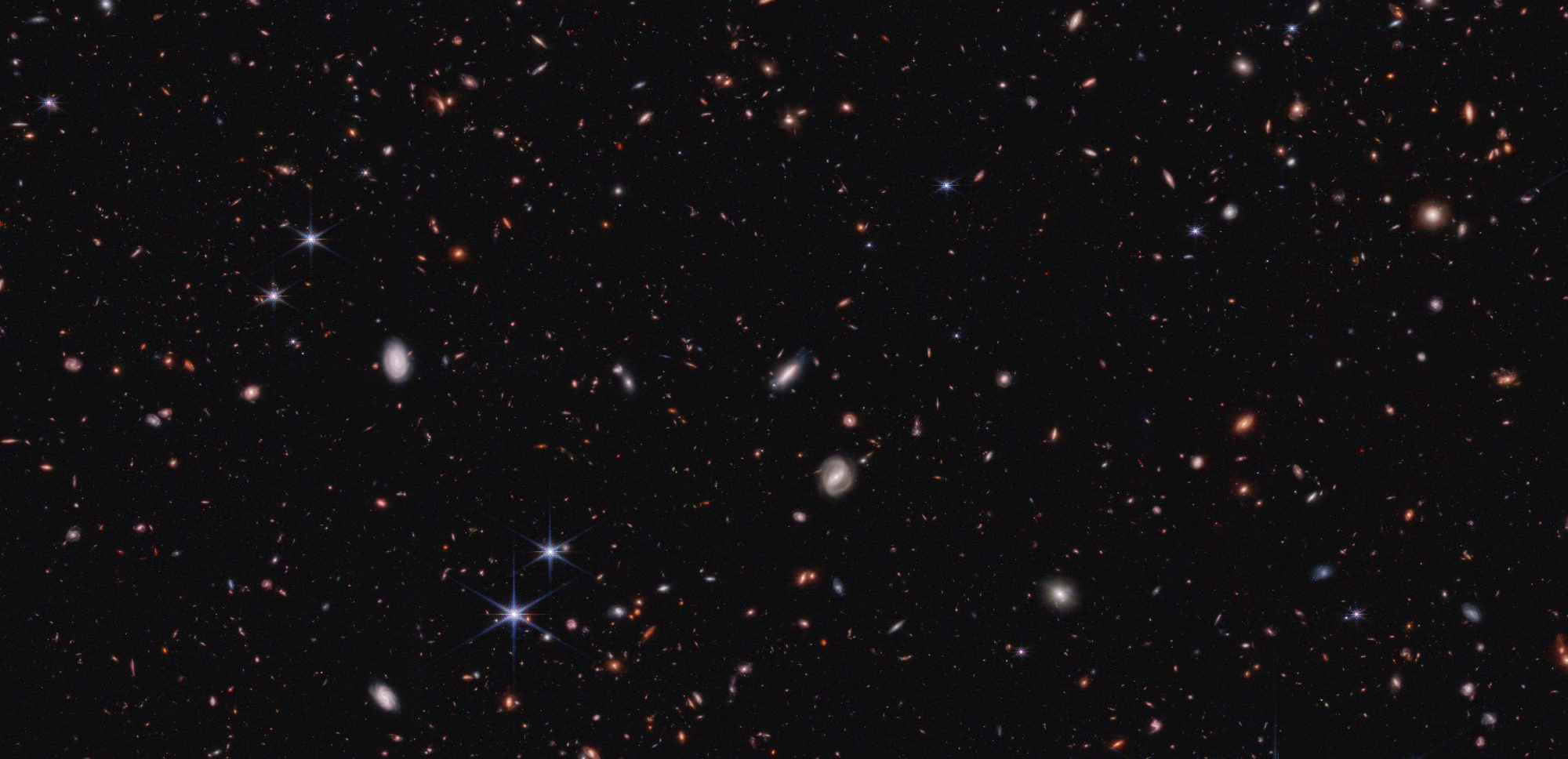
Webb Finds Early Galaxies Weren’t Too Big for Their Britches After All
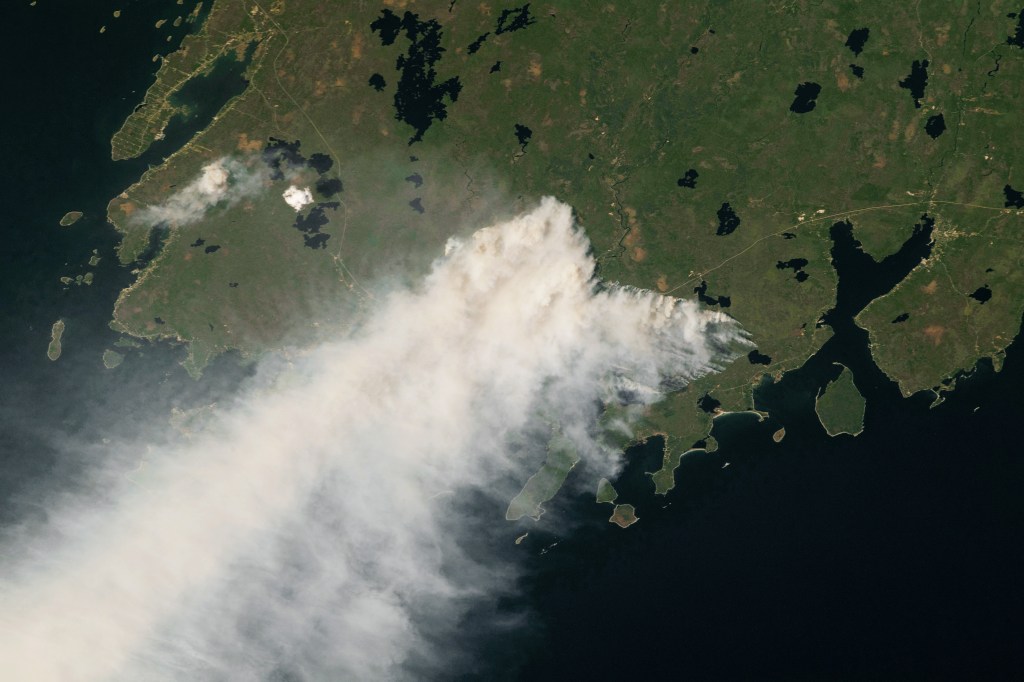
New NASA Study Tallies Carbon Emissions From Massive Canadian Fires
- Search All NASA Missions
- A to Z List of Missions
- Upcoming Launches and Landings
- Spaceships and Rockets
- Communicating with Missions
- James Webb Space Telescope
- Hubble Space Telescope
- Why Go to Space
- Commercial Space
- Destinations
- Living in Space
- Explore Earth Science
- Earth, Our Planet
- Earth Science in Action
- Earth Multimedia
- Earth Science Researchers
- Pluto & Dwarf Planets
- Asteroids, Comets & Meteors
- The Kuiper Belt
- The Oort Cloud
- Skywatching
- The Search for Life in the Universe
- Black Holes
- The Big Bang
- Dark Energy & Dark Matter
- Earth Science
- Planetary Science
- Astrophysics & Space Science
- The Sun & Heliophysics
- Biological & Physical Sciences
- Lunar Science
Citizen Science
- Astromaterials
- Aeronautics Research
- Human Space Travel Research
- Science in the Air
- NASA Aircraft
- Flight Innovation
- Supersonic Flight
- Air Traffic Solutions
- Green Aviation Tech
- Drones & You
- Technology Transfer & Spinoffs
- Space Travel Technology
- Technology Living in Space
- Manufacturing and Materials
- Science Instruments
- For Kids and Students
- For Educators
- For Colleges and Universities
- For Professionals
- Science for Everyone
- Requests for Exhibits, Artifacts, or Speakers
- STEM Engagement at NASA
- NASA's Impacts
- Centers and Facilities
- Directorates
- Organizations
- People of NASA
- Internships
- Our History
- Doing Business with NASA
- Get Involved
NASA en Español
- Aeronáutica
- Ciencias Terrestres
- Sistema Solar
- All NASA News
- Video Series on NASA+
- Newsletters
- Social Media
- Media Resources
- Upcoming Launches & Landings
- Virtual Guest Program
- Image of the Day
- Sounds and Ringtones
- Interactives
- STEM Multimedia
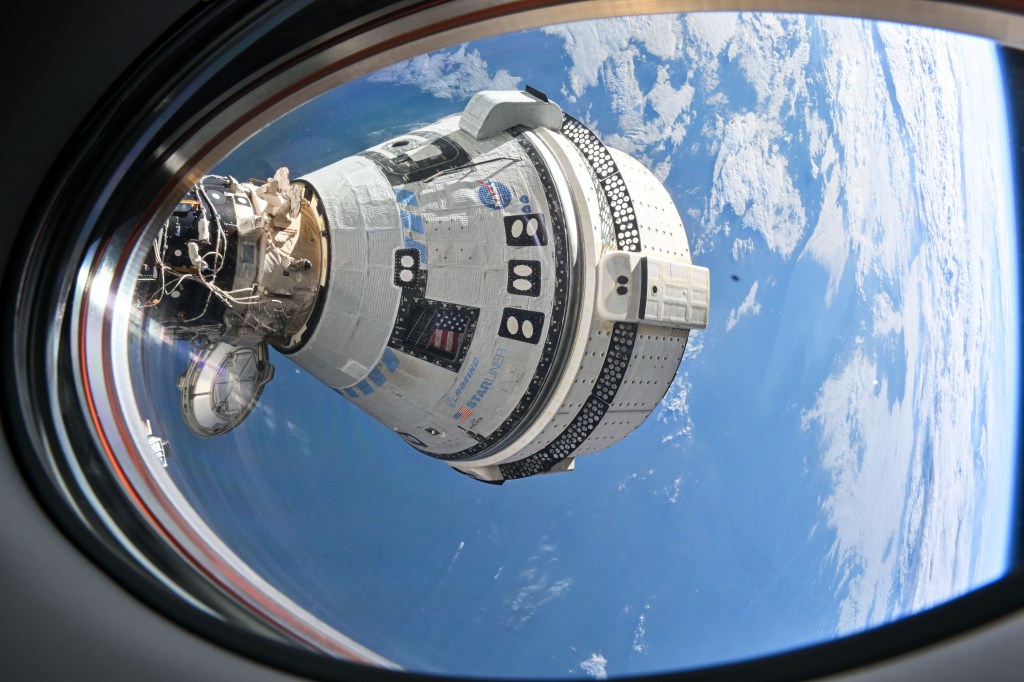
FAQ: NASA’s Boeing Crew Flight Test Return Status
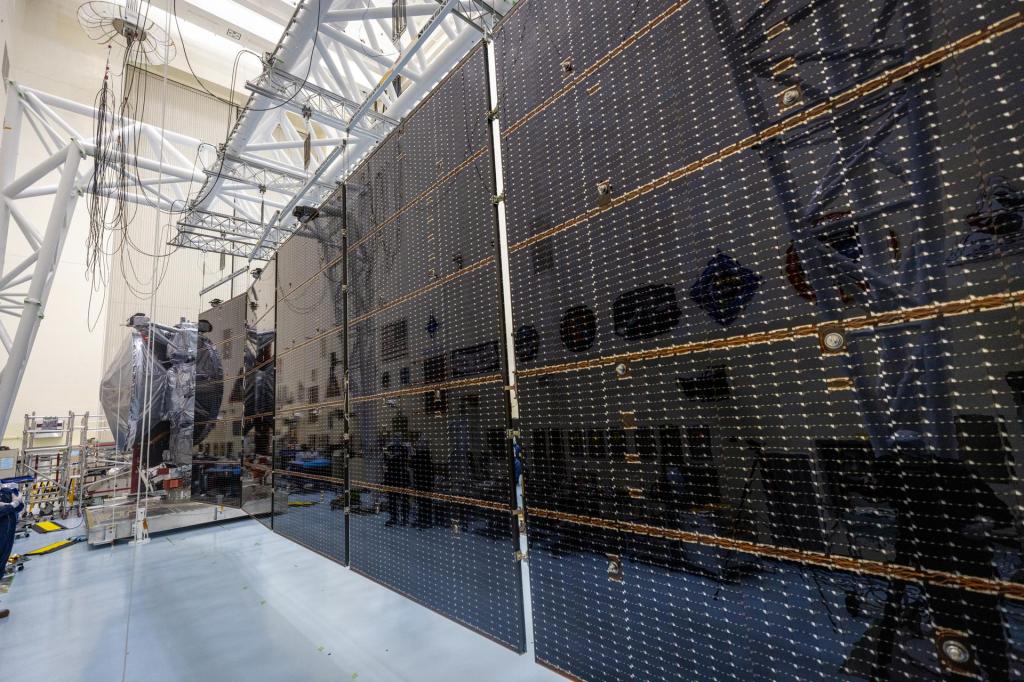
NASA’s Europa Clipper Gets Set of Super-Size Solar Arrays

NASA, Boeing Optimizing Vehicle Assembly Building High Bay for Future SLS Stage Production

NASA Seeks Input for Astrobee Free-flying Space Robots
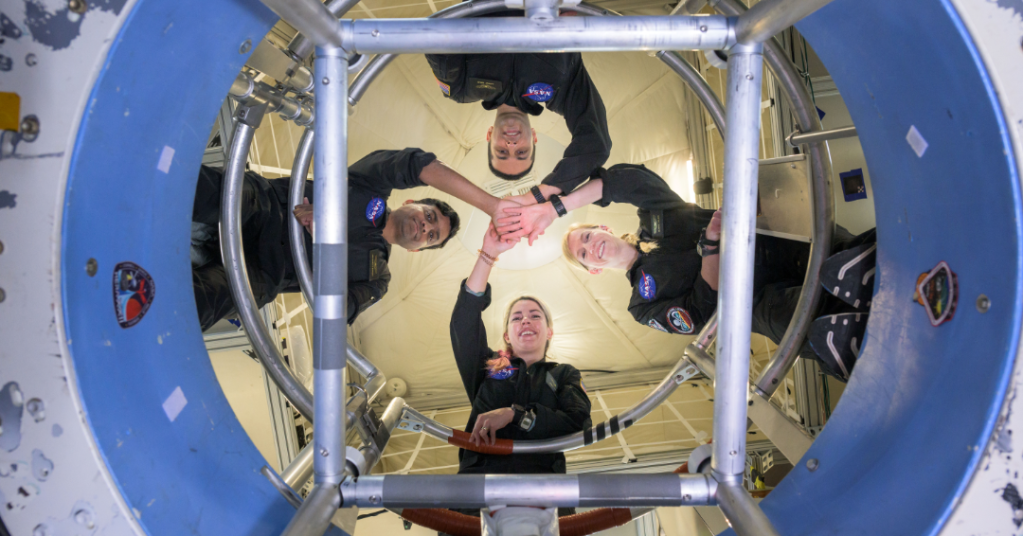
NASA Funds Studies to Support Crew Performance on Long-Duration Missions
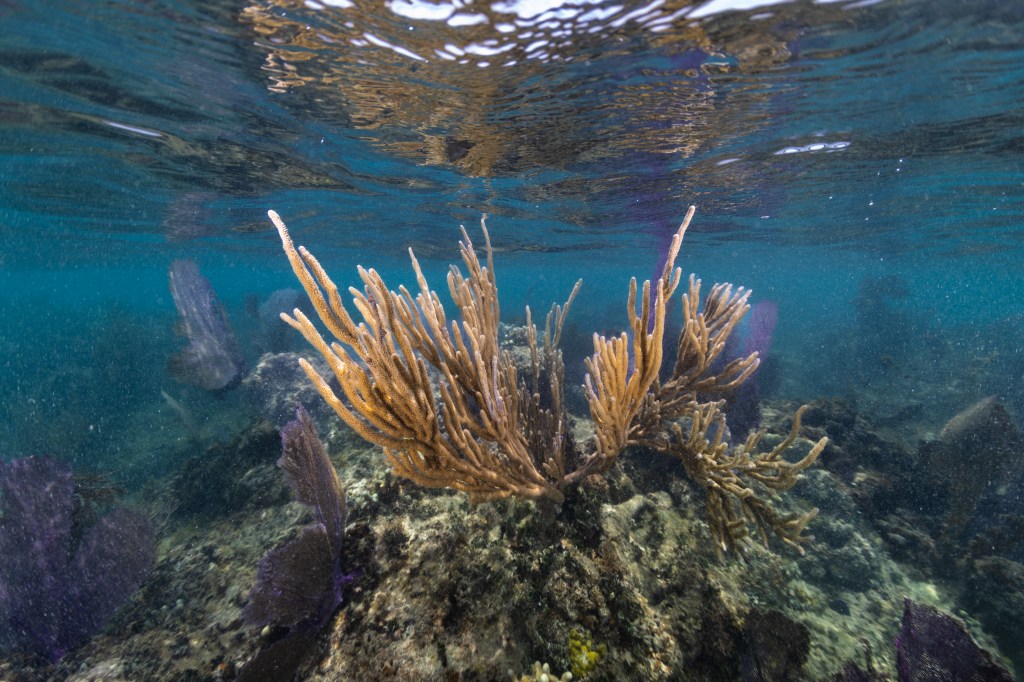
NASA Project in Puerto Rico Trains Students in Marine Biology
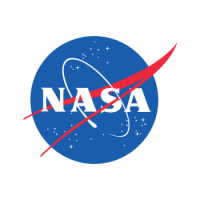
STV Precursor Coincident Datasets
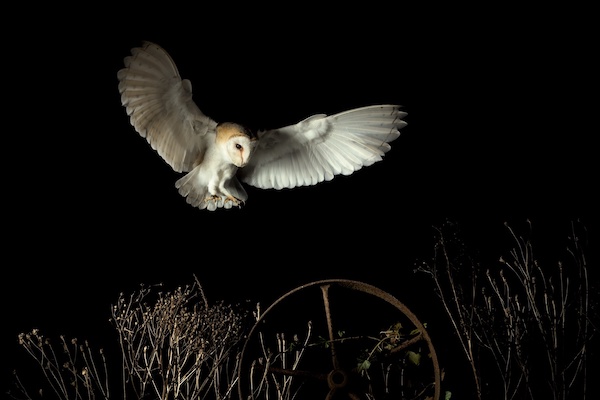
Eclipse Soundscapes AudioMoth Donations Will Study Nature at Night
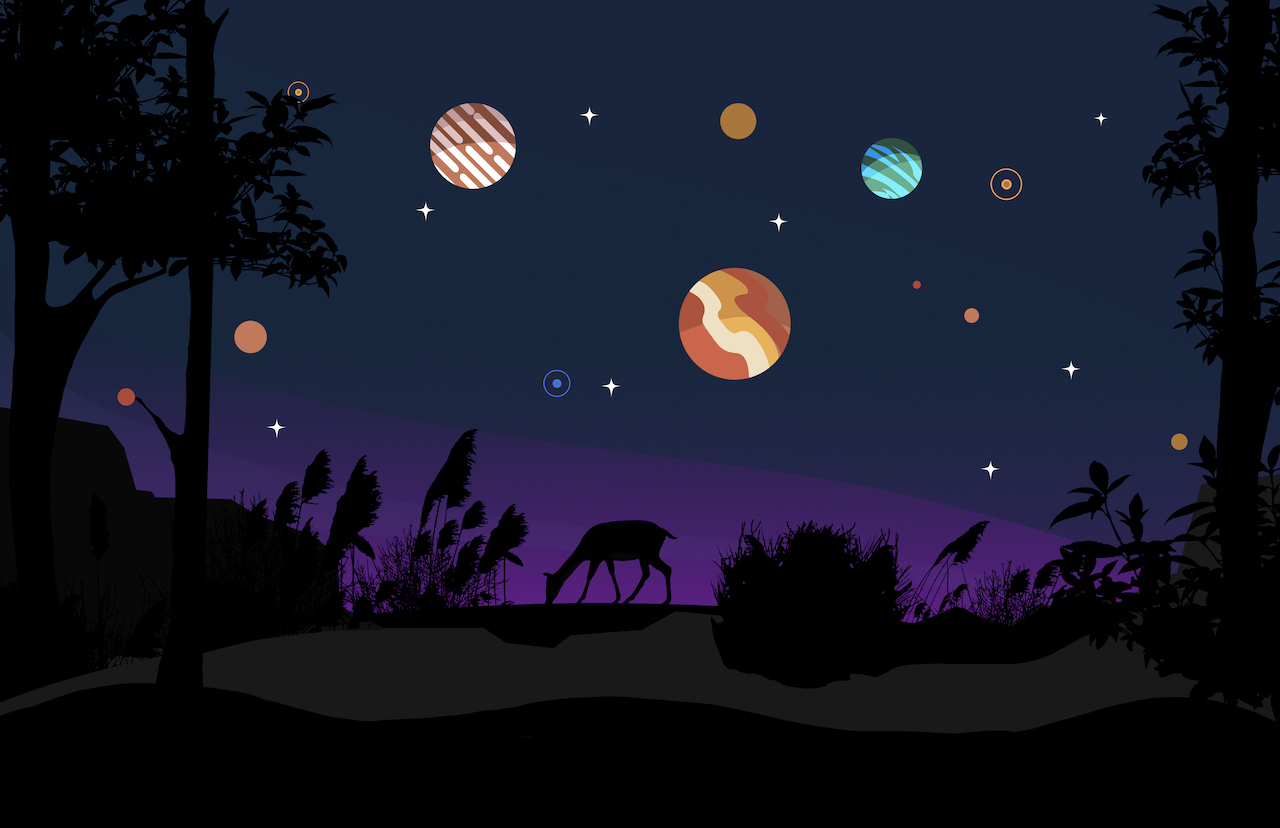
Hubble Traces Star Formation in a Nearby Nebula
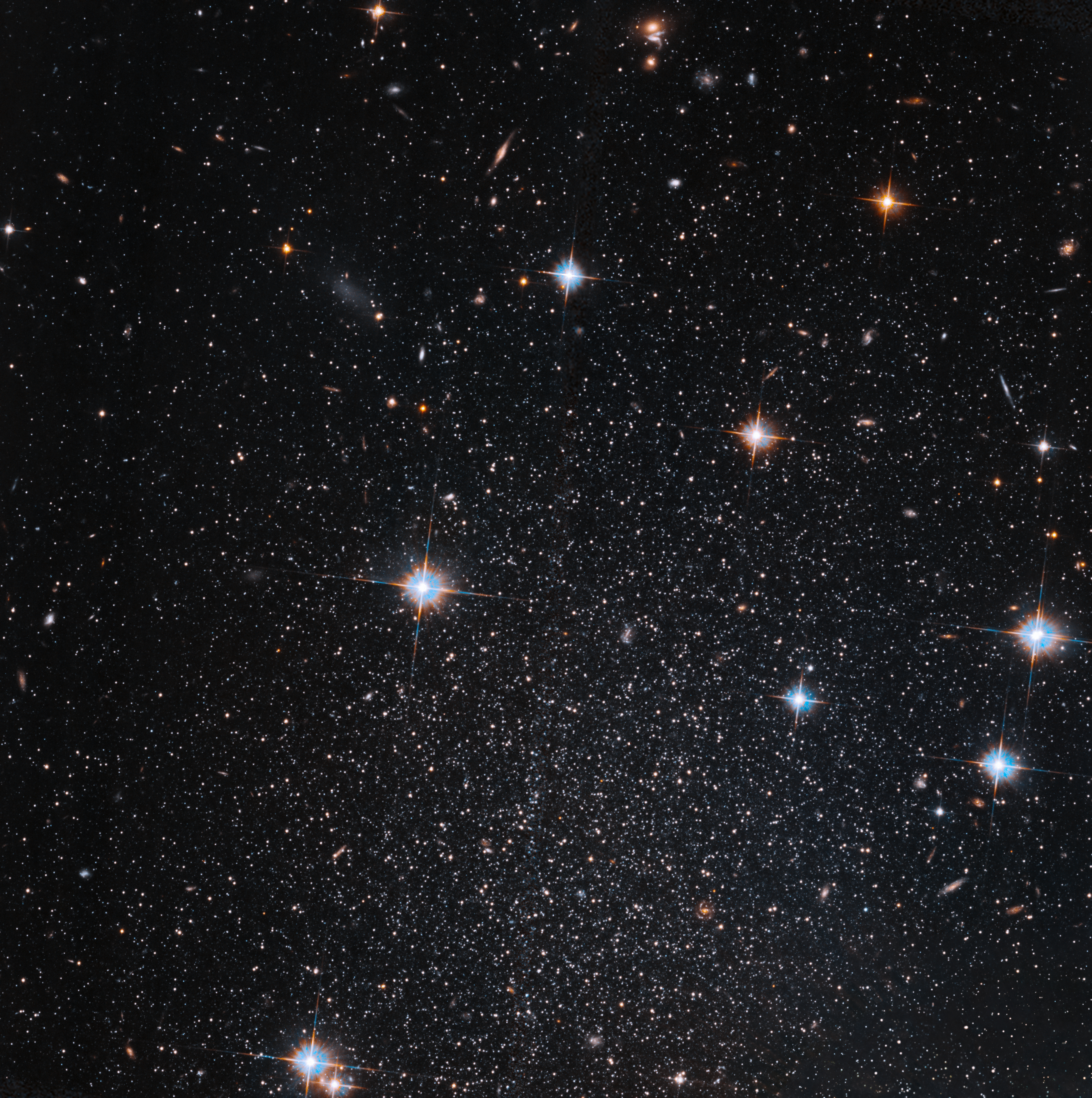
Hubble Pinpoints a Dim, Starry Mini-galaxy
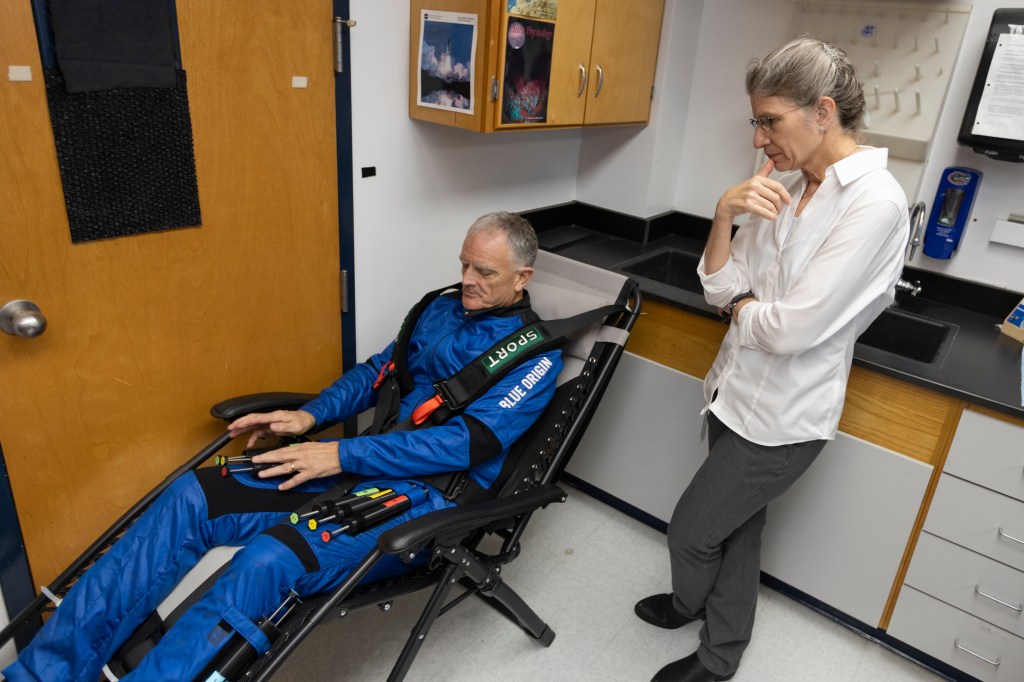
First NASA-Supported Researcher to Fly on Suborbital Rocket
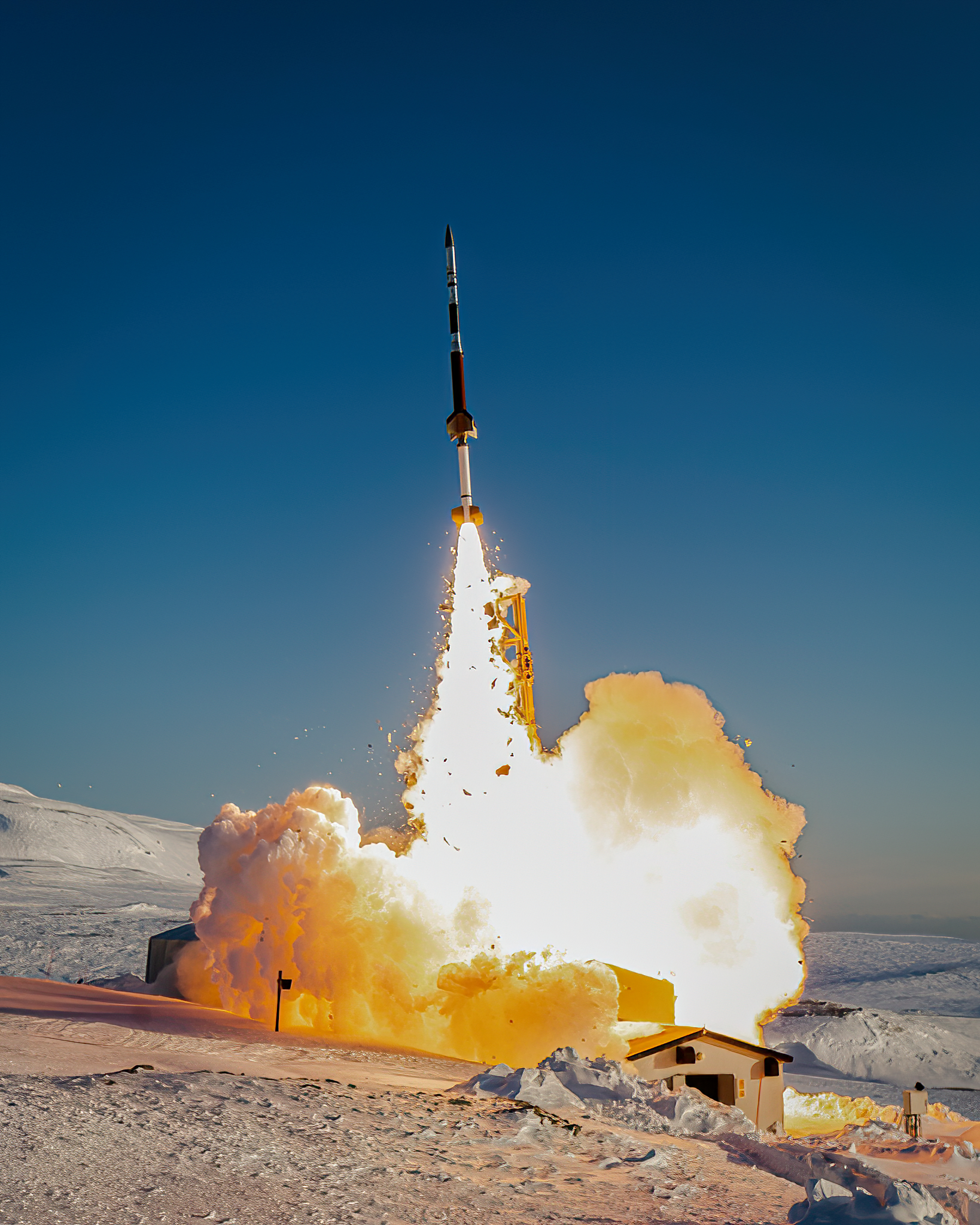
NASA Discovers a Long-Sought Global Electric Field on Earth
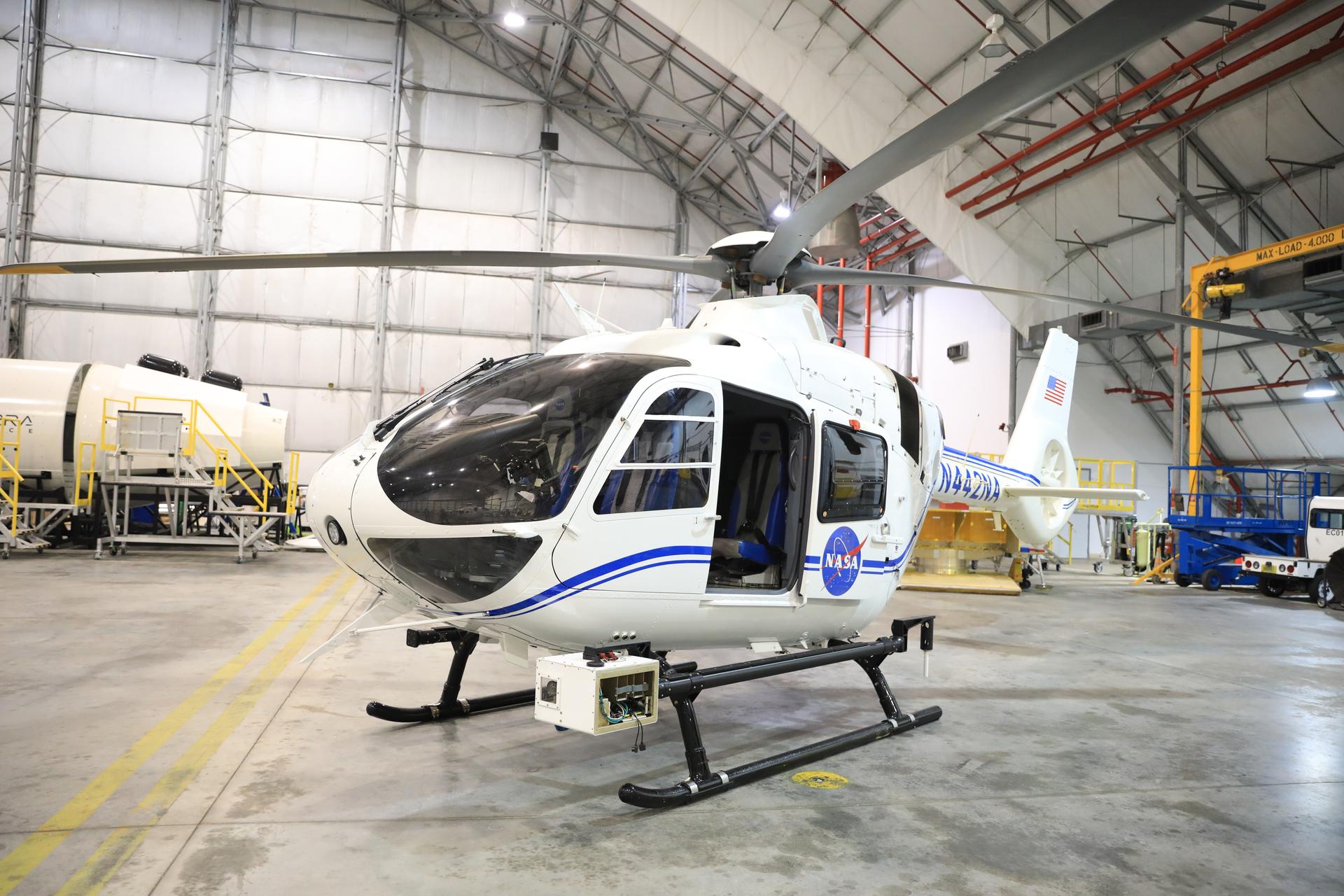
NASA Develops Pod to Help Autonomous Aircraft Operators
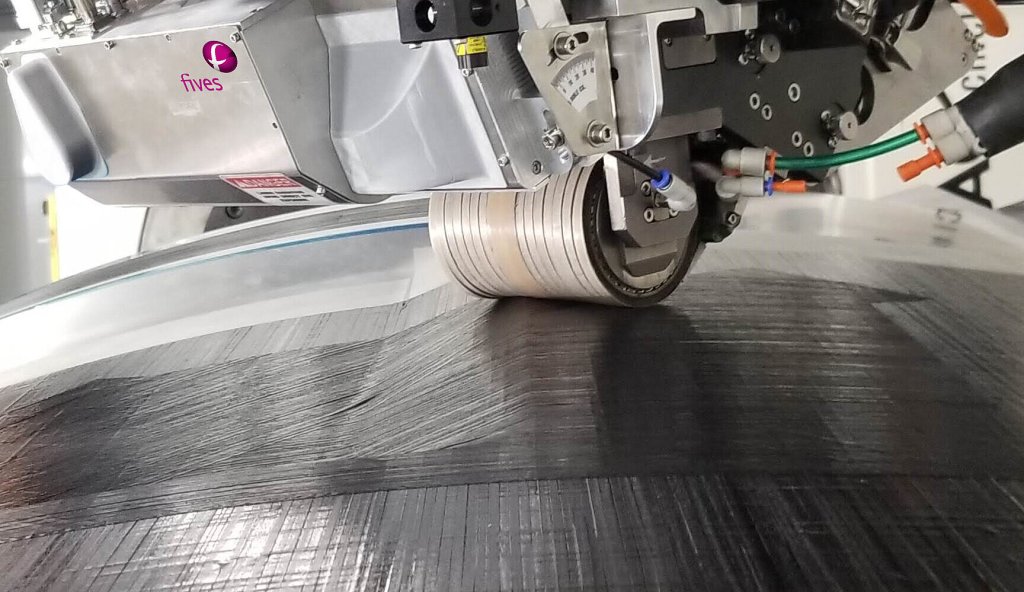
NASA Composite Manufacturing Initiative Gains Two New Members
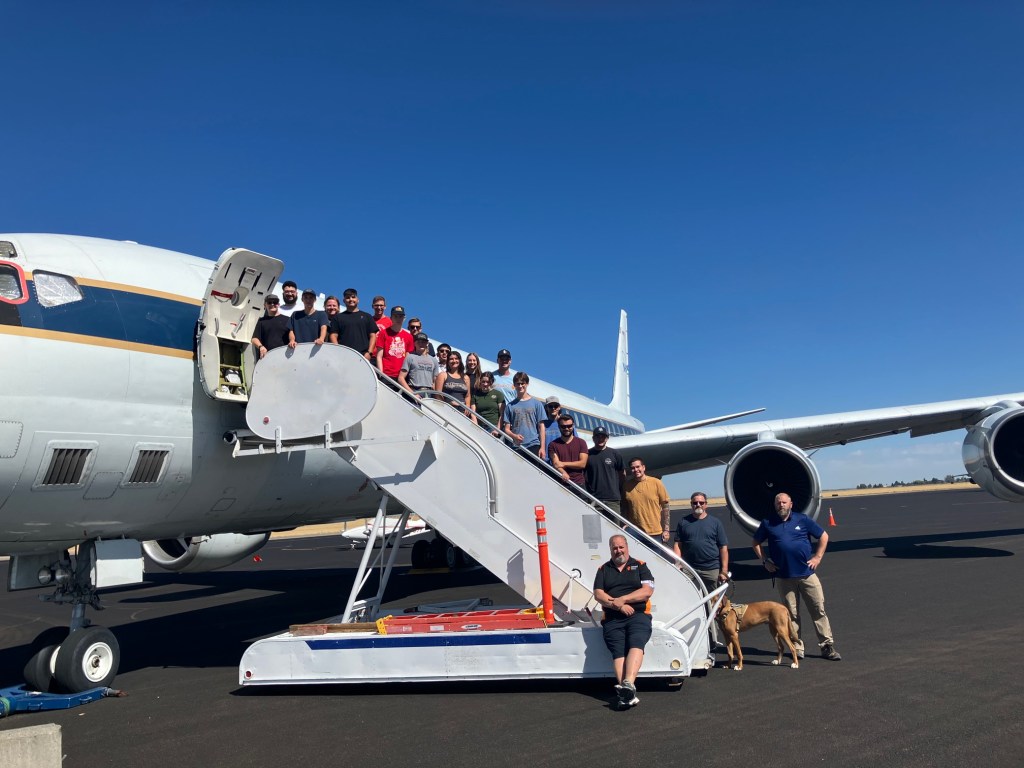
Beyond the Textbook: DC-8 Aircraft Inspires Students in Retirement
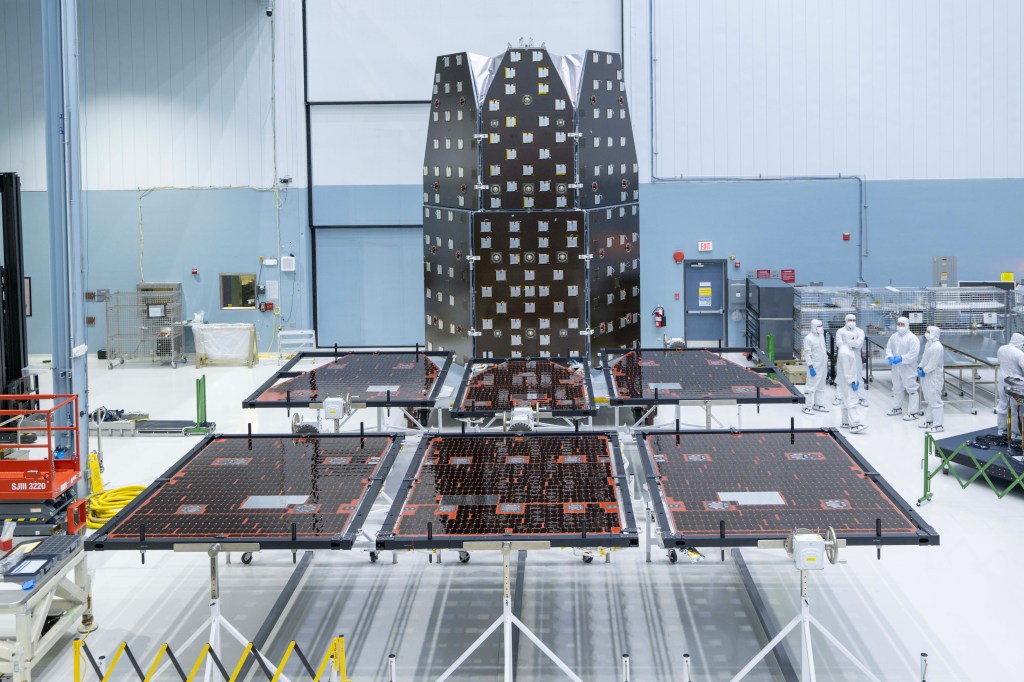
Solar Panels for NASA’s Roman Space Telescope Pass Key Tests
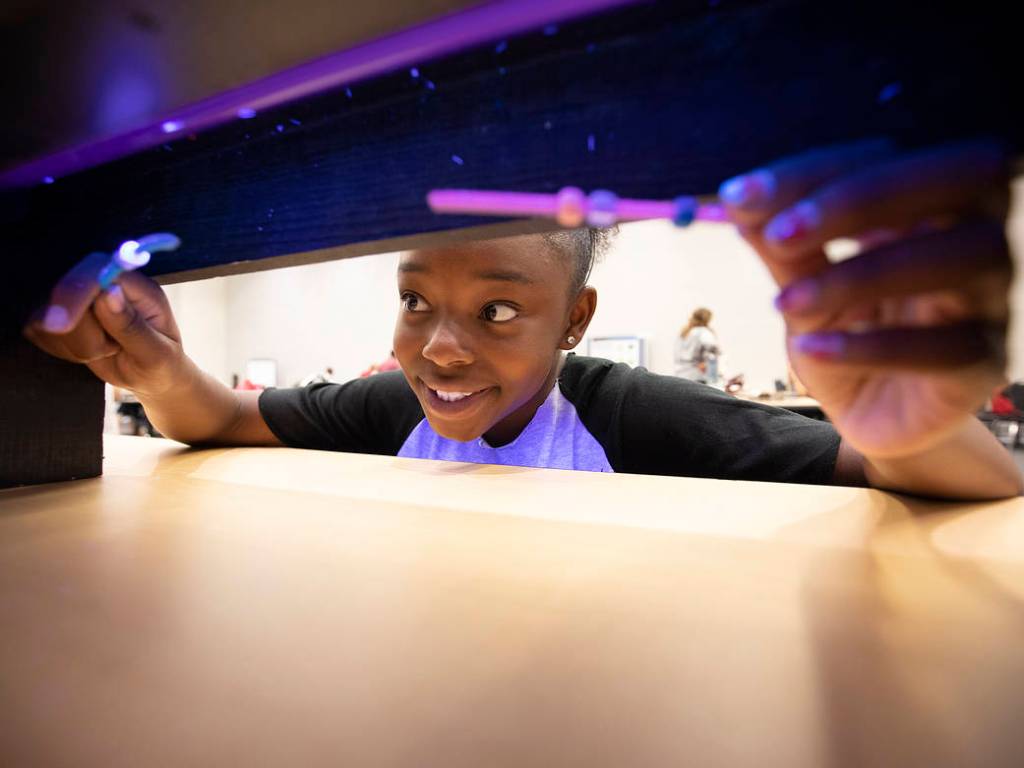
How Do I Navigate NASA Learning Resources and Opportunities?
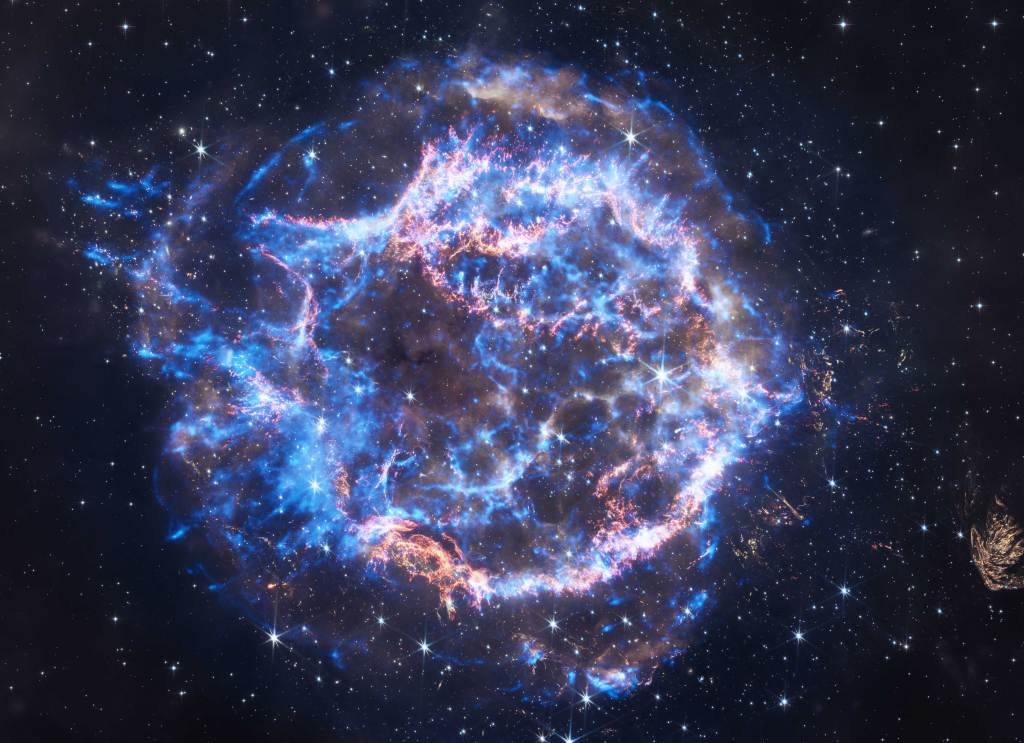
Cassiopeia A, Then the Cosmos: 25 Years of Chandra X-ray Science
Preguntas frecuentes: estado del retorno de la prueba de vuelo tripulado boeing de la nasa.
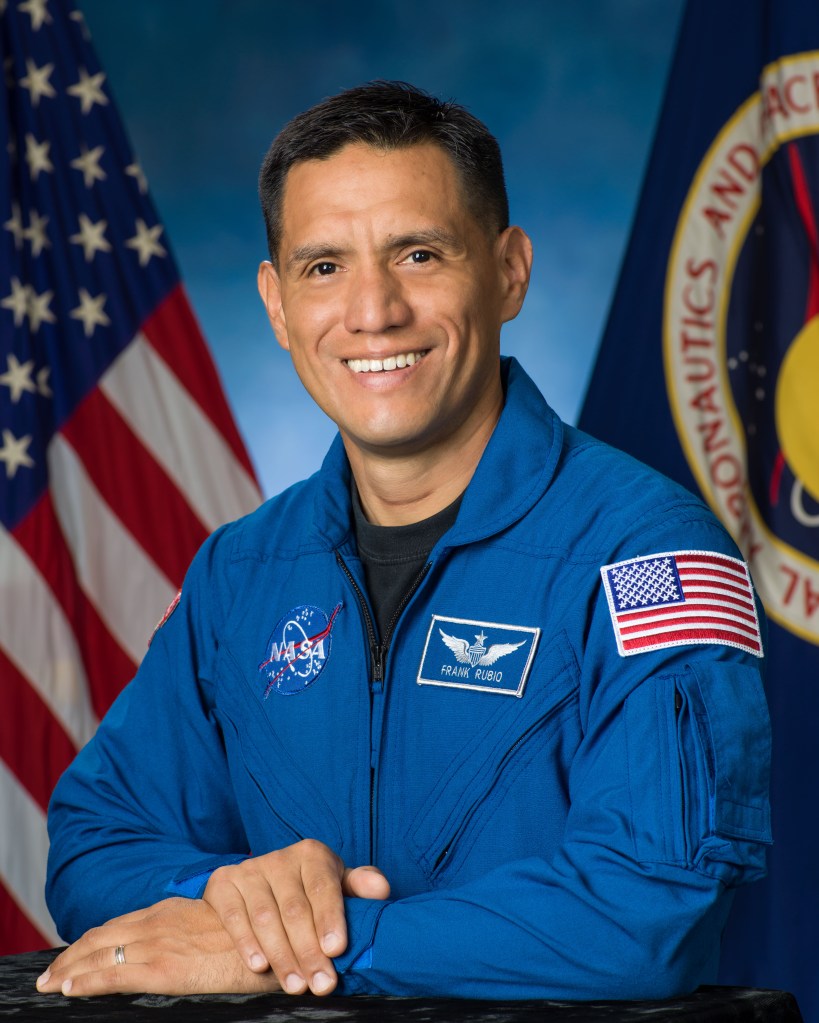
Astronauta de la NASA Frank Rubio
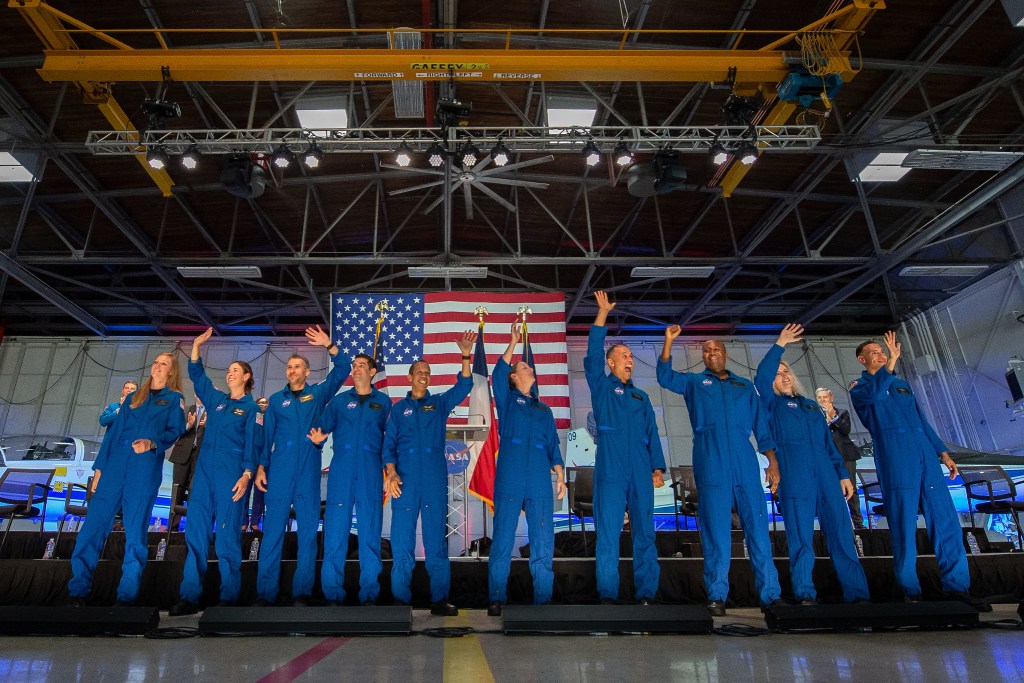
Diez maneras en que los estudiantes pueden prepararse para ser astronautas
Signs of life on mars nasa’s perseverance rover begins the hunt, jet propulsion laboratory, the science team, more about the mission.
After testing a bristling array of instruments on its robotic arm, NASA’s latest Mars rover gets down to business: probing rocks and dust for evidence of past life.
NASA’s Mars 2020 Perseverance rover has begun its search for signs of ancient life on the Red Planet. Flexing its 7-foot (2-meter) mechanical arm, the rover is testing the sensitive detectors it carries, capturing their first science readings. Along with analyzing rocks using X-rays and ultraviolet light, the six-wheeled scientist will zoom in for closeups of tiny segments of rock surfaces that might show evidence of past microbial activity.
Called PIXL , or Planetary Instrument for X-ray Lithochemistry, the rover’s X-ray instrument delivered unexpectedly strong science results while it was still being tested, said Abigail Allwood, PIXL’s principal investigator at NASA’s Jet Propulsion Laboratory in Southern California. Located at the end of the arm, the lunchbox-size instrument fired its X-rays at a small calibration target – used to test instrument settings – aboard Perseverance and was able to determine the composition of Martian dust clinging to the target.
“We got our best-ever composition analysis of Martian dust before it even looked at rock,” Allwood said.
That’s just a small taste of what PIXL, combined with the arm’s other instruments, is expected to reveal as it zeroes in on promising geological features over the weeks and months ahead.
Scientists say Jezero Crater was a crater lake billions of years ago, making it a choice landing site for Perseverance. The crater has long since dried out, and the rover is now picking its way across its red, broken floor .
“If life was there in Jezero Crater, the evidence of that life could be there,” said Allwood, a key member of the Perseverance “arm science” team.
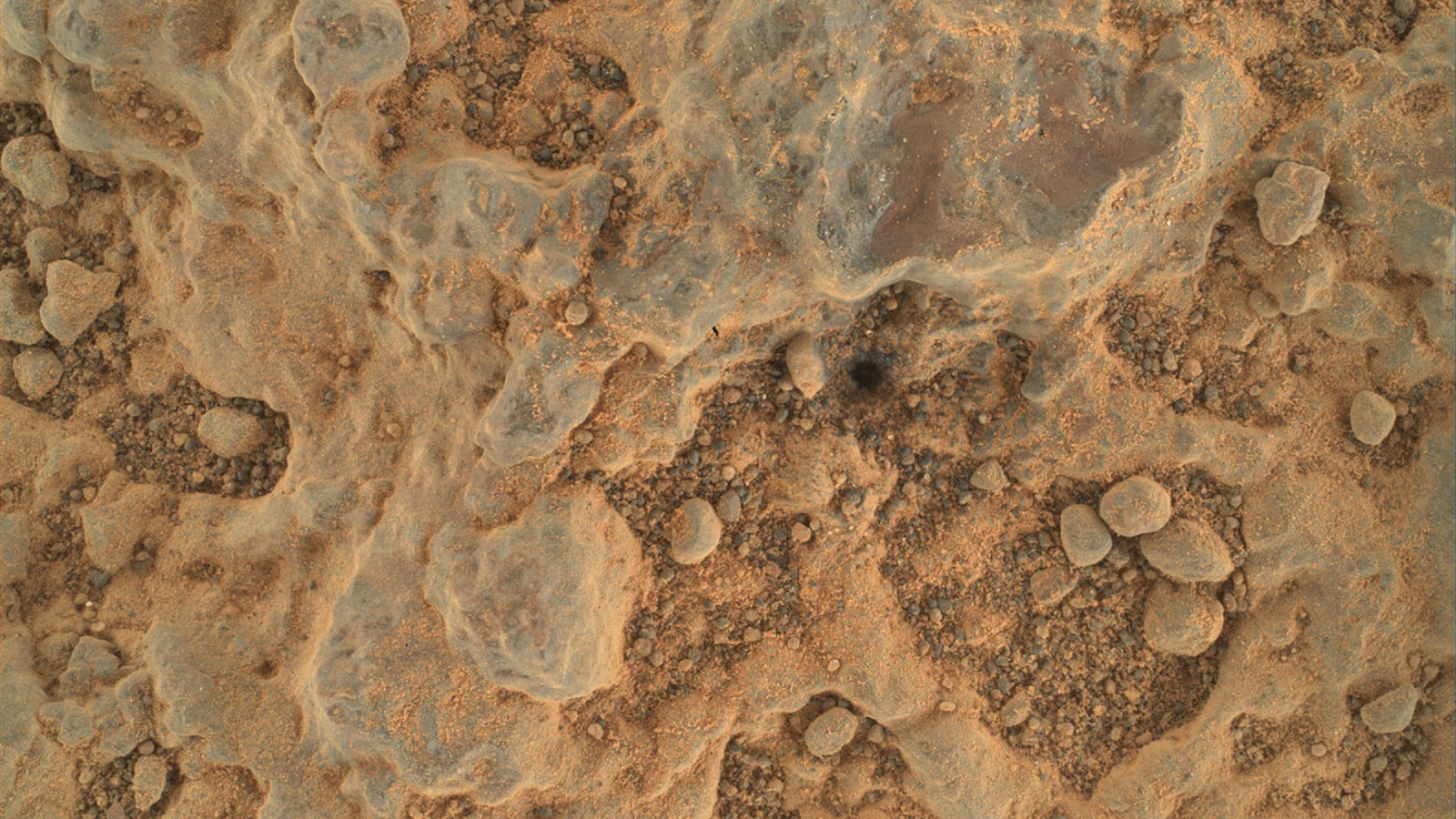
To get a detailed profile of rock textures, contours, and composition, PIXL’s maps of the chemicals throughout a rock can be combined with mineral maps produced by the SHERLOC instrument and its partner, WATSON. SHERLOC – short for Scanning Habitable Environments with Raman & Luminescence for Organics & Chemicals – uses an ultraviolet laser to identify some of the minerals in the rock, while WATSON takes closeup images that scientists can use to determine grain size, roundness, and texture, all of which can help determine how the rock was formed.
Early WATSON closeups have already yielded a trove of data from Martian rocks, the scientists said, such as a variety of colors, sizes of grains in the sediment, and even the presence of “cement” between the grains. Such details can provide important clues about formation history, water flow, and ancient, potentially habitable Martian environments. And combined with those from PIXL, they can provide a broader environmental and even historical snapshot of Jezero Crater.
“What is the crater floor made out of? What were the conditions like on the crater floor?” asks Luther Beegle of JPL, SHERLOC’s principal investigator. “That does tell us a lot about the early days of Mars, and potentially how Mars formed. If we have an idea of what the history of Mars is like, we’ll be able to understand the potential for finding evidence of life.”
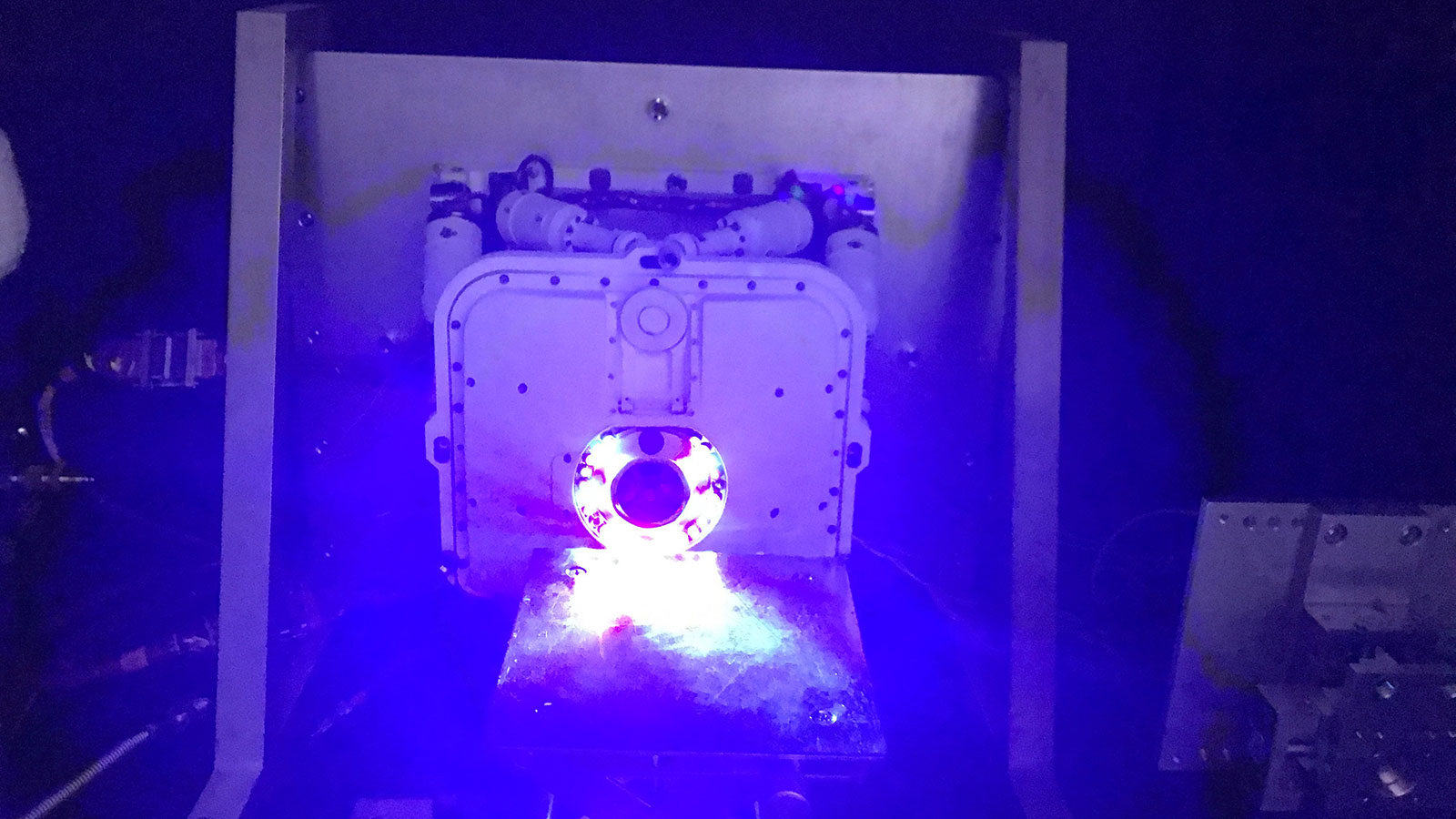
While the rover has significant autonomous capabilities, such as driving itself across the Martian landscape, hundreds of earthbound scientists are still involved in analyzing results and planning further investigations.
“There are almost 500 people on the science team,” Beegle said. “The number of participants in any given action by the rover is on the order of 100. It’s great to see these scientists come to agreement in analyzing the clues, prioritizing each step, and putting together the pieces of the Jezero science puzzle.”
That will be critical when the Mars 2020 Perseverance rover collects its first samples for eventual return to Earth. They’ll be sealed in superclean metallic tubes on the Martian surface so that a future mission could collect them and send back to the home planet for further analysis.
Despite decades of investigation on the question of potential life, the Red Planet has stubbornly kept its secrets.
“Mars 2020, in my view, is the best opportunity we will have in our lifetime to address that question,” said Kenneth Williford, the deputy project scientist for Perseverance.
The geological details are critical, Allwood said, to place any indication of possible life in context, and to check scientists’ ideas about how a second example of life’s origin could come about.
Combined with other instruments on the rover, the detectors on the arm, including SHERLOC and WATSON, could make humanity’s first discovery of life beyond Earth.
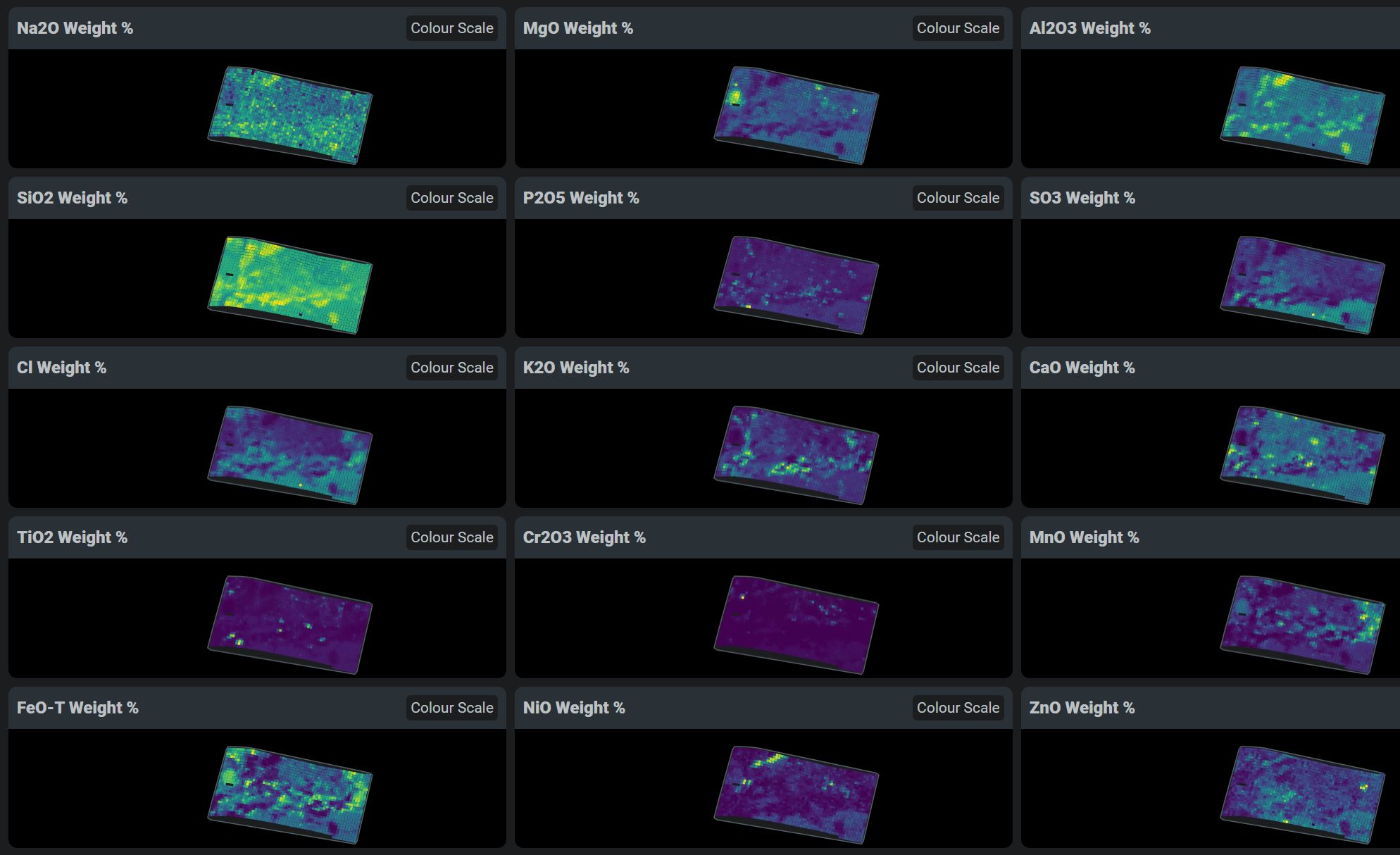
A key objective for Perseverance’s mission on Mars is astrobiology , including the search for signs of ancient microbial life. The rover will characterize the planet’s geology and past climate, pave the way for human exploration of the Red Planet, and be the first mission to collect and cache Martian rock and regolith (broken rock and dust).
Subsequent NASA missions, in cooperation with ESA (European Space Agency), would send spacecraft to Mars to collect these sealed samples from the surface and return them to Earth for in-depth analysis.
The Mars 2020 Perseverance mission is part of NASA’s Moon to Mars exploration approach, which includes Artemis missions to the Moon that will help prepare for human exploration of the Red Planet.
JPL, which is managed for NASA by Caltech in Pasadena, California, built and manages operations of the Perseverance rover.
For more about Perseverance:
mars.nasa.gov/mars2020/
nasa.gov/perseverance
DC Agle Jet Propulsion Laboratory, Pasadena, Calif. 818-393-9011 [email protected]
Karen Fox / Alana Johnson NASA Headquarters, Washington 301-286-6284 / 202-358-1501 [email protected] / [email protected]

IMAGES
VIDEO
COMMENTS
alone on Earth as the only forms of life in the universe. Because of this, research on Mars has been directed at investigating the many different conditions that are conducive to life, especially in early studies. Interestingly, at the start of the 19th century, it was commonly believed that life existed on Mars.
Here are the steps to follow when writing your thesis statement on the research report on life on Mars: Conceptualize your thesis statement. It's always a good idea to brainstorm and have the statement ready before writing. Have the relevant research to help you develop the body of your essay. In this case, read about life on Mars from ...
Reading "Planetary Personhood" in comparison with Space Barons demonstrates then, how posthuman life writing can go beyond simply reproducing of human life and realities on other planets, to rather imagining transformative narratives that question our anthropocentric assumptions.
Searching for evidence of life on Mars is a major impetus for exploration. A new study published in Nature Communications finds that current Mars mission instruments lack the essential sensitivity ...
With the Perseverance rover set to land on Mars on February 18, 2021, we are finally in a position to know where to go, what to look for, and knowing whether there is, or ever was, life on the Red Planet. Science fiction aside, we know that there were not ancient civilizations or a population of little green people on Mars.
This entails many difficult but solvable challenges. However, human activity on Mars depends on the answers to three fundamental questions: (1) Is there life on Mars, does that life represent at second genesis of life? (2) Can Earth life, and humans in particular, adapt to the Martian gravity of 0.38 g, and will human generations on Mars ...
A quest for life on Mars (LoMars) started in the early 1960s when several research articles were published by the most prestigious scientific journals. The rise in annual literature production ...
Life on Mars and the Imagination of Scientists. By Virginia Hughes. December 28, 2012. • 6 min read. Around this time seven years ago I was trying to figure out a topic for my Master's thesis ...
Life On Mars: Past, Present and Future Mars appears to be cold dry and dead world. However there is good evidence that early in its history it had liquid water, more active volcanism, and a thicker atmosphere. Mars had this earth-like environment over three and a half billion years ago, during the same time that life appeared on Earth.
The emphasis on life is well justified; the life-related investigations that are planned range over so much of Mars science that they will result in broad and comprehensive gains in our knowledge; and the areas most neglected as a consequence of this emphasis (see Chapter 12) will, to some extent, be investigated by projected missions of our ...
Tilting toward Life. But Mars goes through cycles of obliquity, or changes in its orbital tilt. Currently, Mars is wobbling back and forth between 15 and 35 degrees' obliquity, on a timescale of about 100,000 years. But every million years or so, it leans over as much as 60 degrees. Along with these changes in obliquity come changes in ...
Searching for life on Mars and its moons. The scientific exploration of Mars over the past several decades has resulted in increasing evidence that the martian surface hosted habitable environments early in its history, as well as evidence of the building blocks of life in the form of organic molecules ( 1 ). Habitats on Mars that could harbor ...
Although the habitability of early Mars is now well established, its suitability for conditions favorable to an independent origin of life (OoL) has been less certain. With continued exploration, evidence has mounted for a widespread diversity of physical and chemical conditions on Mars that mimic those variously hypothesized as settings in which life first arose on Earth. Mars has also ...
After testing a bristling array of instruments on its robotic arm, NASA's latest Mars rover gets down to business: probing rocks and dust for evidence of past life.
If life ever existed on Mars, we may already have the answer at hand. In January NASA's Perseverance rover deposited 10 tubes on the surface of Mars. Each contains a sample of Martian rock that ...
Until that happens, we cannot be sure whether Perseverance has really found fossils of ancient life on Mars. The evidence so far is not definitive, but it is certainly tantalising. NASA Mars Alien ...
The thesis statement of a research report encapsulates the central idea or primary argument of the work. In the context of the research report "Life on Mars," the statement "Some scientists theorized that the seeds for life on Earth were planted by microorganisms from Mars" suggests a specific, scientific hypothesis being explored.
Essay on Life on Mars - Mars is the fourth planet from the sun in our solar system. Also, it is the second smallest planet in our solar system. Possibility of life on mars has aroused the interest of scientists for many years.
To assess Mars' potential for both harboring life and providing useable resources for future human exploration, it is of paramount importance to comprehend the water situation on the planet.
Yesterday, NASA announced that a rock spotted on Mars by its Perseverance rover contained some of the best signs yet that ancient microbial life may have once existed on the Red Planet. The rock provides clear evidence for water, organic matter, and chemical reactions that could provide an energy source, Laurie Leshin, director of the Jet ...
Crafting a thesis statement about Mars is challenging and requires extensive research and critical thinking. Key aspects to consider include scientific exploration, potential colonization, environmental conditions, and the search for extraterrestrial life. Staying up-to-date on the latest discoveries is also important given the dynamic nature of the field. While complex, seeking expert ...
However, there's evidence that billions of years ago, the thicker atmosphere allowed liquid water to persist on the surface of the Red Planet. Since liquid water is essential for life, scientists want to know if life emerged on Mars and search for evidence of ancient Martian life by examining Mars rocks for organic molecules such as amino acids.
A key objective for Perseverance's mission on Mars is astrobiology, including the search for signs of ancient microbial life. The rover will characterize the planet's geology and past climate, pave the way for human exploration of the Red Planet, and be the first mission to collect and cache Martian rock and regolith (broken rock and dust).
The Gallo-Roman enclosure of Le Mans, also referred to as the Roman enclosure of Le Mans, is located in the city once known as Vindinum or Vindunum.This city was the capital of the Gallic people, the Aulerci Cenomani.The construction of the enclosure began during the Late Roman Empire.While it was previously believed to date from the end of the 3rd century, recent studies suggest a ...