- Search Menu
- Sign in through your institution
- Advance Articles
- Author Guidelines
- Call for Papers
- Submission Site
- Open Access Options
- Self-Archiving Policy
- Publish with us
- About Oxford Open Climate Change
- Editorial Board
- Advertising & Corporate Services
- Journals on Oxford Academic
- Books on Oxford Academic


Article Contents
Background information and structure of paper, climate sensitivity (ecs and ess), climate response time, cenozoic era, perspective on policy implications, acknowledgements, supplementary data, conflict of interest, data availability, authors’ contributions.
- < Previous
Global warming in the pipeline
- Article contents
- Figures & tables
- Supplementary Data
James E Hansen, Makiko Sato, Leon Simons, Larissa S Nazarenko, Isabelle Sangha, Pushker Kharecha, James C Zachos, Karina von Schuckmann, Norman G Loeb, Matthew B Osman, Qinjian Jin, George Tselioudis, Eunbi Jeong, Andrew Lacis, Reto Ruedy, Gary Russell, Junji Cao, Jing Li, Global warming in the pipeline, Oxford Open Climate Change , Volume 3, Issue 1, 2023, kgad008, https://doi.org/10.1093/oxfclm/kgad008
- Permissions Icon Permissions
Improved knowledge of glacial-to-interglacial global temperature change yields Charney (fast-feedback) equilibrium climate sensitivity 1.2 ± 0.3°C (2σ) per W/m 2 , which is 4.8°C ± 1.2°C for doubled CO 2 . Consistent analysis of temperature over the full Cenozoic era—including ‘slow’ feedbacks by ice sheets and trace gases—supports this sensitivity and implies that CO 2 was 300–350 ppm in the Pliocene and about 450 ppm at transition to a nearly ice-free planet, exposing unrealistic lethargy of ice sheet models. Equilibrium global warming for today’s GHG amount is 10°C, which is reduced to 8°C by today’s human-made aerosols. Equilibrium warming is not ‘committed’ warming; rapid phaseout of GHG emissions would prevent most equilibrium warming from occurring. However, decline of aerosol emissions since 2010 should increase the 1970–2010 global warming rate of 0.18°C per decade to a post-2010 rate of at least 0.27°C per decade. Thus, under the present geopolitical approach to GHG emissions, global warming will exceed 1.5°C in the 2020s and 2°C before 2050. Impacts on people and nature will accelerate as global warming increases hydrologic (weather) extremes. The enormity of consequences demands a return to Holocene-level global temperature. Required actions include: (1) a global increasing price on GHG emissions accompanied by development of abundant, affordable, dispatchable clean energy, (2) East-West cooperation in a way that accommodates developing world needs, and (3) intervention with Earth’s radiation imbalance to phase down today’s massive human-made ‘geo-transformation’ of Earth’s climate. Current political crises present an opportunity for reset, especially if young people can grasp their situation.
It has been known since the 1800s that infrared-absorbing (greenhouse) gases (GHGs) warm Earth’s surface and that the abundance of GHGs changes naturally as well as from human actions [ 1 , 2 ]. 1 Roger Revelle wrote in 1965 that we are conducting a ‘vast geophysical experiment’ by burning fossil fuels that accumulated in Earth’s crust over hundreds of millions of years [ 3 ] Carbon dioxide (CO 2 ) in the air is now increasing and already has reached levels that have not existed for millions of years, with consequences that have yet to be determined. Jule Charney led a study in 1979 by the United States National Academy of Sciences that concluded that doubling of atmospheric CO 2 was likely to cause global warming of 3 ± 1.5°C [ 4 ]. Charney added: ‘However, we believe it is quite possible that the capacity of the intermediate waters of the ocean to absorb heat could delay the estimated warming by several decades.’
After U.S. President Jimmy Carter signed the 1980 Energy Security Act, which included a focus on unconventional fossil fuels such as coal gasification and rock fracturing (‘fracking’) to extract shale oil and tight gas, the U.S. Congress asked the National Academy of Sciences again to assess potential climate effects. Their massive Changing Climate report had a measured tone on energy policy—amounting to a call for research [ 5 ]. Was not enough known to caution lawmakers against taxpayer subsidy of the most carbon-intensive fossil fuels? Perhaps the equanimity was due in part to a major error: the report assumed that the delay of global warming caused by the ocean’s thermal inertia is 15 years, independent of climate sensitivity. With that assumption, they concluded that climate sensitivity for 2 × CO 2 is near or below the low end of Charney’s 1.5–4.5°C range. If climate sensitivity was low and the lag between emissions and climate response was only 15 years, climate change would not be nearly the threat that it is.
Simultaneous with preparation of Changing Climate , climate sensitivity was addressed at the 1982 Ewing Symposium at the Lamont Doherty Geophysical Observatory of Columbia University on 25–27 October, with papers published in January 1984 as a monograph of the American Geophysical Union [ 6 ]. Paleoclimate data and global climate modeling together led to an inference that climate sensitivity is in the range 2.5–5°C for 2 × CO 2 and that climate response time to a forcing is of the order of a century, not 15 years [ 7 ]. Thus, the concept that a large amount of additional human-made warming is already ‘in the pipeline’ was introduced. E.E. David, Jr, President of Exxon Research and Engineering, in his keynote talk at the symposium insightfully noted [ 8 ]: ‘The critical problem is that the environmental impacts of the CO 2 buildup may be so long delayed. A look at the theory of feedback systems shows that where there is such a long delay, the system breaks down, unless there is anticipation built into the loop.’
Thus, the danger caused by climate’s delayed response and the need for anticipatory action to alter the course of fossil fuel development was apparent to scientists and the fossil fuel industry 40 years ago. 2 Yet industry chose to long deny the need to change energy course [ 9 ], and now, while governments and financial interests connive, most industry adopts a ‘greenwash’ approach that threatens to lock in perilous consequences for humanity. Scientists will share responsibility if we allow governments to rely on goals for future global GHG levels, as if targets had meaning in the absence of policies required to achieve them.
The Intergovernmental Panel on Climate Change (IPCC) was established in 1988 to provide scientific assessments on the state of knowledge about climate change [ 10 ] and almost all nations agreed to the 1992 United Nations Framework Convention on Climate Change [ 11 ] with the objective to avert ‘dangerous anthropogenic interference with the climate system’. The current IPCC Working Group 1 report [ 12 ] provides a best estimate of 3°C for equilibrium global climate sensitivity to 2 × CO 2 and describes shutdown of the overturning ocean circulations and large sea level rise on the century time scale as ‘high impact, low probability’ even under extreme GHG growth scenarios. This contrasts with ‘high impact, high probability’ assessments reached in a paper [ 13 ]—hereafter abbreviated Ice Melt—that several of us published in 2016. Recently, our paper’s first author (JEH) described a long-time effort to understand the effect of ocean mixing and aerosols on observed and projected climate change, which led to a conclusion that most climate models are unrealistically insensitive to freshwater injected by melting ice and that ice sheet models are unrealistically lethargic in the face of rapid, large climate change [ 14 ].
Eelco Rohling, editor of Oxford Open Climate Change, invited a perspective article on these issues. Our principal motivation in this paper is concern that IPCC has underestimated climate sensitivity and understated the threat of large sea level rise and shutdown of ocean overturning circulations, but these issues, because of their complexity, must be addressed in two steps. Our present paper addresses climate sensitivity and warming in the pipeline, concluding that these exceed IPCC’s best estimates. Response of ocean circulation and ice sheet dynamics to global warming—already outlined in the Ice Melt paper—will be addressed further in a later paper.
The structure of our present paper is as follows. Climate sensitivity section makes a fresh evaluation of Charney’s equilibrium climate sensitivity (ECS) based on improved paleoclimate data and introduces Earth system sensitivity (ESS), which includes the feedbacks that Charney held fixed. Climate response time section explores the fast-feedback response time of Earth’s temperature and energy imbalance to an imposed forcing, concluding that cloud feedbacks buffer heat uptake by the ocean, thus increasing the delay in surface warming and making Earth’s energy imbalance an underestimate of the forcing reduction required to stabilize climate. Cenozoic era section analyzes temperature change of the past 66 million years and infers the Cenozoic history of CO 2 , thus providing insights about climate change. Aerosols section addresses the absence of aerosol forcing data via inferences from paleo data and modern global temperature change, and we point out potential information in ‘the great inadvertent aerosol experiment’ provided by recent restrictions on fuels in international shipping. Summary section discusses policy implications of high climate sensitivity and the delayed response of the climate system. Warming in the pipeline need not appear. We can take actions that slow and reverse global warming; indeed, we suggest that such actions are needed to avoid disastrous consequences for humanity and nature. Reduction of greenhouse gas emissions as rapidly as practical has highest priority, but that policy alone is now inadequate and must be complemented by additional actions to affect Earth’s energy balance. The world is still early in this ‘vast geophysical experiment’—as far as consequences are concerned—but time has run short for the ‘anticipation’ that E.E. David recommended.
This section gives a brief overview of the history of ECS estimates since the Charney report and uses glacial-to-interglacial climate change to infer an improved estimate of ECS. We discuss how ECS and the more general Earth system sensitivity (ESS) depend on the climate state.
Charney defined ECS as the eventual global temperature change caused by doubled CO 2 if ice sheets, vegetation and long-lived GHGs are fixed (except the specified CO 2 doubling). Other quantities affecting Earth’s energy balance—clouds, aerosols, water vapor, snow cover and sea ice—change rapidly in response to climate change. Thus, Charney’s ECS is also called the ‘fast-feedback’ climate sensitivity. Feedbacks interact in many ways, so their changes are calculated in global climate models (GCMs) that simulate such interactions. Charney implicitly assumed that change of the ice sheets on Greenland and Antarctica—which we categorize as a ‘slow feedback’—was not important on time scales of most public interest.
ECS defined by Charney is a gedanken concept that helps us study the effect of human-made and natural climate forcings. If knowledge of ECS were based only on models, it would be difficult to narrow the range of estimated climate sensitivity—or have confidence in any range—because we do not know how well feedbacks are modeled or if the models include all significant real-world feedbacks. Cloud and aerosol interactions are complex, e.g. and even small cloud changes can have a large effect. Thus, data on Earth’s paleoclimate history are essential, allowing us to compare different climate states, knowing that all feedbacks operated.
Climate sensitivity estimated at the 1982 Ewing Symposium
We evaluated contributions of individual feedback processes to g by inserting changes of water vapor, clouds, and surface albedo (reflectivity, literally whiteness, due to sea ice and snow changes) from the 2 × CO 2 GCM simulation one-by-one into a one-dimensional radiative-convective model [ 16 ], finding g wv = 0.4, g cl = 0.2, g sa = 0.1, where g wv , g cl , and g sa are the water vapor, cloud and surface albedo gains. The 0.2 cloud gain was about equally from a small increase in cloud top height and a small decrease in cloud cover. These feedbacks all seemed reasonable, but how could we verify their magnitudes or the net ECS due to all feedbacks?
We recognized the potential of emerging paleoclimate data. Early data from polar ice cores revealed that atmospheric CO 2 was much less during glacial periods and the CLIMAP project [ 17 ] used proxy data to reconstruct global surface conditions during the Last Glacial Maximum (LGM), which peaked about 20 000 years ago. A powerful constraint was the fact that Earth had to be in energy balance averaged over the several millennia of the LGM. However, when we employed CLIMAP boundary conditions including sea surface temperatures (SSTs), Earth was out of energy balance, radiating 2.1 W/m 2 to space, i.e. Earth was trying to cool off with an enormous energy imbalance, equivalent to half of 2 × CO 2 forcing.
Something was wrong with either assumed LGM conditions or our climate model. We tried CLIMAP’s maximal land ice—this only reduced the energy imbalance from 2.1 to 1.6 W/m 2 . Moreover, we had taken LGM CO 2 as 200 ppm and did not know that CH 4 and N 2 O were less in the LGM than in the present interglacial period; accurate GHGs and CLIMAP SSTs produce a planetary energy imbalance close to 3 W/m 2 . Most feedbacks in our model were set by CLIMAP. Sea ice is set by CLIMAP. Water vapor depends on surface temperature, which is set by CLIMAP SSTs. Cloud feedback is uncertain, but ECS smaller than 2.4°C for 2 × CO 2 would require a negative cloud gain. g cl ∼ 0.2 from our GCM increases ECS from 2.4°C to 4°C ( Equation 1 ) and accounts for almost the entire difference of sensitivities of our model (4°C for 2 × CO 2 ) and the Manabe and Stouffer model [ 18 ] (2°C for 2 × CO 2 ) that had fixed cloud cover and cloud height. Manabe suggested [ 19 ] that our higher ECS was due to a too-large sea ice and snow feedback, but we noted [ 7 ] that sea ice in our control run was less than observed, so we likely understated sea ice feedback. Amplifying feedback due to high clouds increasing in height with warming is expected and is found in observations, large-eddy simulations and GCMs [ 20 ] Sherwood et al . [ 21 ] conclude that negative low-cloud feedback is ‘neither credibly suggested by any model, nor by physical principles, nor by observations.’ Despite a wide spread among models, GCMs today show an amplifying cloud feedback due to increases in cloud height and decreases in cloud amount, despite increases in cloud albedo [ 22 ]. These cloud changes are found in all observed cloud regimes and locations, implying robust thermodynamic control [ 23 ].
CLIMAP SSTs were a more likely cause of the planetary energy imbalance. Co-author D. Peteet used pollen data to infer LGM tropical and subtropical cooling 2–3°C greater than in a GCM forced by CLIMAP SSTs. D. Rind and Peteet found that montane LGM snowlines in the tropics descended 1 km in the LGM, inconsistent with climate constrained by CLIMAP SSTs. CLIMAP assumed that tiny shelled marine species migrate to stay in a temperature zone they inhabit today. But what if, instead, these species partly adapt over millennia to changing temperature? Based on the work of Rind and Peteet, later published [ 24 ], we suspected but could not prove that CLIMAP SSTs were too warm.
Based on GCM simulations for 2 × CO 2 , on our feedback analysis for the LGM, and on observed global warming in the past century, we concluded that ECS was in the range 2.5–5°C for 2 × CO 2 . If CLIMAP SSTs were accurate, ECS was near the low end of that range. In contrast, our analysis implied that ECS for 2 × CO 2 was in the upper half of the 2.5–5°C range, but our analysis depended in part on our GCM, which had sensitivity 4°C for 2 × CO 2 . To resolve the matter, a paleo thermometer independent of biologic adaptation was needed. Several decades later, such a paleo thermometer and advanced analysis techniques exist. We will use recent studies to infer our present best estimates for ECS and ESS. First, however, we will comment on other estimates of climate sensitivity and clarify the definition of climate forcings that we employ.
IPCC and independent climate sensitivity estimates
Reviews of climate sensitivity are available, e.g. Rohling et al . [ 25 ], which focuses on the physics of the climate system, and Sherwood et al . [ 26 ], which adds emphasis on probabilistic combination of multiple uncertainties. Progress in narrowing the uncertainty in climate sensitivity was slow in the first five IPCC assessment reports. The fifth assessment report [ 26 ] (AR5) in 2014 concluded only—with 66% probability—that ECS was in the range 1.5–4.5°C, the same as Charney’s report 35 years earlier. The broad spectrum of information on climate change—especially constraints imposed by paleoclimate data—at last affected AR6 [ 12 ], which concluded with 66% probability that ECS is 2.5–4°C, with 3°C as their best estimate ( Supplementary Fig. TS.6 ).
Sherwood et al . [ 21 ] combine three lines of evidence: climate feedback studies, historical climate change, and paleoclimate data, inferring S = 2.6–3.9°C with 66% probability for 2 × CO 2 , where S is an ‘effective sensitivity’ relevant to a 150-year time scale. They find ECS only slightly larger: 2.6–4.1°C with 66% probability. Climate feedback studies, inherently, cannot yield a sharp definition of ECS, as we showed in the cloud feedback discussion above. Earth’s climate system includes amplifying feedbacks that push the gain, g, closer to unity than zero, thus making ECS sensitive to uncertainty in any feedback; the resulting sensitivity of ECS to g prohibits precise evaluation from feedback analysis. Similarly, historical climate change cannot define ECS well because the aerosol climate forcing is unmeasured. Also, forced and unforced ocean dynamics give rise to a pattern effect: [ 27 ] the geographic pattern of transient and equilibrium temperature changes differ, which affects ECS inferred from transient climate change. These difficulties help explain how Sherwood et al . [ 21 ] could estimate ECS as only 6% larger than S , an implausible result in view of the ocean’s great thermal inertia. An intercomparison of GCMs run for millennial time scales, LongRunMIP [ 28 ], includes 14 simulations of 9 GCMs with runs of 5000 years (or close enough for extrapolation to 5000 years). Their global warmings at 5000 years range from 30% to 80% larger than their 150-year responses.
Our approach is to compare glacial and interglacial equilibrium climate states. The change of atmospheric and surface forcings can be defined accurately, thus leading to a sharp evaluation of ECS for cases in which equilibrium response is assured. With this knowledge in hand, additional information can be extracted from historical and paleo climate changes.
Climate forcing definitions
Attention to climate forcing definitions is essential for quantitative analysis of climate change. However, readers uninterested in radiative forcings may skip this section with little penalty. We describe our climate forcing definition and compare our forcings with those of IPCC. Our total GHG forcing matches that of IPCC within a few percent, but this close fit hides larger differences in individual forcings that deserve attention.
A further refinement of climate forcing is suggested in Efficacy : effective forcing (F e ) defined by a long GCM run with calculated ocean temperature. The resulting global surface temperature change, relative to that for equal CO 2 forcing, defines the forcing’s efficacy. Effective forcings, F e , were found to be within a few percent of F s for most forcing agents, i.e. the results confirm that F s is a robust forcing. This support is for F s , not for F o = ERF, which is systematically smaller than F s . The Goddard Institute for Space Studies (GISS) GCM [ 32 , 33 ] used for CMIP6 [ 34 ] studies, which we label the GISS (2020) model, 3 has higher resolution (2° × 2.5° and 40 atmospheric layers) and other changes that yield a moister upper troposphere and lower stratosphere, relative to the GISS model used in Efficacy . GHG forcings reported for the GISS (2020) model [ 32 , 33 ] are smaller than in prior GISS models, a change attributed [ 33 ] to blanketing by high level water vapor. However, part of the change is from comparison of F o in GISS (2020) to F S in earlier models. The 2 × CO 2 fixed SST simulation with the GISS (2020) model yields F o = 3.59 W/m 2 , δTo = 0.27°C and λ = 0.9°C per W/m 2 . Thus F S = 3.59 + 0.30 = 3.89 W/m 2 , which is only 5.4% smaller than the F S = 4.11 W/m 2 for the GISS model used in Efficacy .
Greenhouse gas radiative forcings
Gas . | Radiative Forcing . |
---|---|
CO | F = f(c) – f(c ), where f(c) = 4.996 ln (c + 0.0005c ) |
CH | F = 0.0406(√m − √m ) − [g(m, n ) – g(m , n )] |
N O | F = 0.136(√n – √n ) – [g(m , n) – g(m , n )] |
where g(m, n) = 0.5 ln [1 + 2 × 10 (mn) ] | |
CFC-11 | F = 0.264(x − x ) |
CFC-12 | F = 0.323(y − y ) |
Gas . | Radiative Forcing . |
---|---|
CO | F = f(c) – f(c ), where f(c) = 4.996 ln (c + 0.0005c ) |
CH | F = 0.0406(√m − √m ) − [g(m, n ) – g(m , n )] |
N O | F = 0.136(√n – √n ) – [g(m , n) – g(m , n )] |
where g(m, n) = 0.5 ln [1 + 2 × 10 (mn) ] | |
CFC-11 | F = 0.264(x − x ) |
CFC-12 | F = 0.323(y − y ) |
c, CO 2 (ppm); m, CH 4 (ppb); n, N 2 O (ppb); x/y, CFC-11/12 (ppb).
The CH 4 coefficient (1.45) includes the effect of CH 4 on O 3 and stratospheric H 2 O, as well as the efficacy (1.10) of CH 4 per se . We assume that CH 4 is responsible for 45% of the O 3 change [ 37 ]. Forcing caused by the remaining 55% of the O 3 change is based on IPCC AR6 O 3 forcing (Fa = 0.47 W/m 2 in 2019); we multiply this AR6 O 3 forcing by 0.55 × 0.82 = 0.45, where 0.82 is the efficacy of O 3 forcing from Table 1 of Efficacy . Thus, the non-CH 4 portion of the O 3 forcing is 0.21 W/m 2 in 2019. MPTGs and OTGs are Montreal Protocol Trace Gases and Other Trace Gases [ 38 ]. A list of these gases and a table of annual forcings since 1992 are available as well as the earlier data [ 39 ].
The climate forcing from our formulae is slightly larger than IPCC AR6 forcings ( Fig. 1 ). In 2019, the final year of AR6 data, our GHG forcing is 4.00 W/m 2 ; the AR6 forcing is 3.84 W/m 2 . Our forcing should be larger, because IPCC forcings are F o for all gases except O 3 , for which they provide F a (AR6 section 7.3.2.5). Table 1 in Efficacy allows accurate comparison: δT o for 2 × CO 2 for the GISS model used in Efficacy is 0.22°C, λ is 0.67°C per W/m 2 , so δT o /λ = 0.33 W/m 2 . Thus, the conversion factor from F o to F e (or F s ) is 4.11/(4.11−0.33). The non-O 3 portion of AR6 2019 forcing (3.84−0.47 = 3.37) W/m 2 increases to 3.664 W/m 2 . The O 3 portion of the AR6 2019 forcing (0.47 W/m 2 ) decreases to 0.385 W/m 2 because the efficacy of F a (O 3 ) is 0.82. The AR6 GHG forcing in 2019 is thus ∼4.05 W/m 2 , expressed as Fe ∼ Fs, which is ∼1% larger than follows from our formulae. This precise agreement is not indicative of the true uncertainty in the GHG forcing, which IPCC AR6 estimates as 10%, thus about 0.4 W/m 2 . We concur with their error estimate and employ it in our ECS uncertainty analysis (Equilibrium climate sensitivity section).
![abstract for global warming research paper IPCC AR6 Annex III greenhouse gas forcing [12], which employs Fa for O3 and Fo for other GHGs, compared with the effective forcing, Fe, from Equation (4). See discussion in text.](https://oup.silverchair-cdn.com/oup/backfile/Content_public/Journal/oocc/3/1/10.1093_oxfclm_kgad008/1/m_kgad008f1.jpeg?Expires=1726813424&Signature=pxyjfK39E0Q1lN955dU6BuoTYdLy5kInIEqfY9IyV9PkXzOuhVYxmaqgIeQMuny34Fs2PTsePG~YqDfkgPjqSiPk6PAjRdrA30DHiC7wQ1grgA855zyfmHxlUbhvmviLsbhocBj0AfGB9qVBwHMBdacVxSDePnYavVTLzoHtZz16uHC8BlTJzLc0GpmyFZH4IN7L9uRU0Q~Nb8Znr~t40geGuvrKx1UjhlDLMIqqvA~s~nZdtEIT06r6vGYe9Sc1LBDlTlVnnnSGohy0jBMfGTbSUA0Hh1YBMZYom8UTdGjhcpa0dc6h2~LH7oZExNoMOFsEPCYtzADl74nmz072yg__&Key-Pair-Id=APKAIE5G5CRDK6RD3PGA)
IPCC AR6 Annex III greenhouse gas forcing [ 12 ], which employs F a for O 3 and F o for other GHGs, compared with the effective forcing, F e , from Equation (4) . See discussion in text.
We conclude that the GHG increase since 1750 already produces a climate forcing equivalent to that of 2 × CO 2 (our formulae yield F e ∼ F s = 4.08 W/m 2 for 2021 and 4.13 W/m 2 for 2022; IPCC AR6 has F s = 4.14 W/m 2 for 2021). The human-made 2 × CO 2 climate forcing imagined by Charney, Tyndall and other greenhouse giants is no longer imaginary. Humanity is now taking its first steps into the period of consequences. Earth’s paleoclimate history helps us assess the potential outcomes.
Glacial-to-interglacial climate oscillations
In this section we describe how ice core data help us assess ECS for climate states from glacial conditions to interglacial periods such as the Holocene, the interglacial period of the past 12 000 years. We discuss climate sensitivity in warmer climates in Cenozoic era section.
Air bubbles in Antarctic ice cores—trapped as snow piled up and compressed into ice—preserve a record of long-lived GHGs for at least 800 000 years. Isotopic composition of the ice provides a measure of temperature in and near Antarctica [ 40 ]. Changes of temperature and CO 2 are highly correlated ( Fig. 2 ). This does not mean that CO 2 is the primal cause of the climate oscillations. Hays et al . [ 42 ] showed that small changes of Earth’s orbit and the tilt of Earth’s spin axis are pacemakers of the ice ages. Orbital changes alter the seasonal and geographical distribution of insolation, which affects ice sheet size and GHG amount. Long-term climate is sensitive because ice sheets and GHGs act as amplifying feedbacks: [ 43 ] as Earth warms, ice sheets shrink, expose a darker surface, and absorb more sunlight; also, as Earth warms, the ocean and continents release GHGs to the air. These amplifying feedbacks work in the opposite sense as Earth cools. Orbital forcings oscillate slowly over tens and hundreds of thousands of years [ 44 ]. The picture of how Earth orbital changes drive millennial climate change was painted in the 1920s by Milutin Milankovitch, who built on 19th century hypotheses of James Croll and Joseph Adhémar. Paleoclimate changes of ice sheets and GHGs are sometimes described as slow feedbacks [ 45 ], but their slow change is paced by the Earth orbital forcing; their slow change does not mean that these feedbacks cannot operate more rapidly in response to a rapid climate forcing.
![abstract for global warming research paper Antarctic Dome C temperature for past 800 ky from Jouzel et al. [40] relative to the mean of the last 10 ky and Dome C CO2 amount from Luthi et al. [41] (kyBP is kiloyears before present).](https://oup.silverchair-cdn.com/oup/backfile/Content_public/Journal/oocc/3/1/10.1093_oxfclm_kgad008/1/m_kgad008f2.jpeg?Expires=1726813425&Signature=ISbaixqHzImSna2AVJ6UZ-VsI3OBQRGgxmmphT9qVb86W~9Vq-nlN-5ZPT-m7njEg~HCs871p6056NwJOh9iDgP9FiWD~~hNYhr~jXDkM9L420fSsU0ztWt7OJqDxHACxe-dISYNXWLoyr~ayAkwYB1JXuxIbv4CgJeY2euKD~Kp7HhY16EuLoHBqW1HqLVU8RrM8HI6VXQGr01TAAPOr33x0cnBJLEp~MKCL-vgFise3dxpm9jgv43wk1HUMd9cF-0pGvB7dvoxBtkJW1bfepctFktWjQSthRgPnr6FJKqGjIQwINdtkBuahbPxj-zMH-BLI7YUq9y7zkOVcfXTIA__&Key-Pair-Id=APKAIE5G5CRDK6RD3PGA)
Antarctic Dome C temperature for past 800 ky from Jouzel et al . [ 40 ] relative to the mean of the last 10 ky and Dome C CO 2 amount from Luthi et al . [ 41 ] (kyBP is kiloyears before present).
We evaluate ECS by comparing stable climate states before and after a glacial-to-interglacial climate transition. GHG amounts are known from ice cores and ice sheet sizes are known from geologic data. This empirical ECS applies to the range of global temperature covered by ice cores, which we will conclude is about –7°C to + 1°C relative to the Holocene. The Holocene is an unusual interglacial. Maximum melt rate was at 13.2 kyBP, as expected [ 45 ] and GHG amounts began to decline after peaking early in the Holocene, as in most interglacials. However, several ky later, CO 2 and CH 4 increased, raising a question of whether humans were affecting GHGs. Ruddiman [ 46 ] suggests that deforestation began to affect CO 2 6500 years ago and rice irrigation began to affect CH 4 5000 years ago. Those possibilities complicate use of LGM-Holocene warming to estimate ECS. However, sea level, and thus the size of the ice sheets, had stabilized by 7000 years ago (Evidence of aerosol forcing in the Holocene section). Thus, the millennium centered on 7 kyBP provides a good period to compare with the LGM. Comparison of the Eemian interglacial ( Fig. 2 ) with the prior glacial maximum (PGM) has potential for independent assessment.
LGM-Holocene and PGM-Eemian evaluation of ECS
In this section we evaluate ECS by comparing neighboring glacial and interglacial periods when Earth was in energy balance within less than 0.1 W/m 2 averaged over a millennium. Larger imbalance would cause temperature or sea level change that did not occur [ 48 ]. 4 Thus, we can assess ECS from knowledge of atmospheric and surface forcings that maintained these climates.
Recent advanced analysis techniques allow improved estimate of paleo temperatures. Tierney et al . [ 49 ] exclude microbiology fossils whose potential to adapt makes them dubious thermometers. Instead, they use a large collection of geochemical (isotope) proxies for SST in an analysis constrained by climate change patterns defined by GCMs. They find cooling of 6.1°C (95% confidence: 5.7–6.5°C) for the interval 23–19 kyBP. A similarly constrained global analysis by Osman et al . [ 50 ] finds LGM cooling at 21–18 kyBP of 7.0 ± 1°C (95% confidence). Tierney (priv. comm.) attributes the difference between the two studies to the broader time interval of the former study, and concludes that peak LGM cooling was near 7°C.
Seltzer et al . [ 51 ] use the temperature-dependent solubility of dissolved noble gases in ancient groundwater to show that land areas between 45°S and 35°N cooled 5.8 ± 0.6°C in the LGM. This cooling is consistent with 1 km lowering of alpine snowlines found by Rind and Peteet [ 24 ]. Land response to a forcing exceeds ocean response, but polar amplification makes the global response as large as the low latitude land response in GCM simulations with fixed ice sheets ( Supplementary Material Fig. S3 ). When ice sheet growth is added, cooling amplification at mid and high latitudes is greater [ 7 ], making 5.8°C cooling of low latitude land consistent with global cooling of ∼7°C.
LGM CO 2 , CH 4 and N 2 O amounts are known accurately with the exception of N 2 O in the PGM when N 2 O reactions with dust in the ice core corrupt the data. We take PGM N 2 O as the mean of the smallest reported PGM amount and the LGM amount; potential error in the N 2 O forcing is ∼0.01 W/m 2 . We calculate CO 2 , CH 4 , and N 2 O forcings using Equation (4) and formulae for each gas in Supplementary Material for the periods shown by green bars in Fig. 3 . The Eemian period avoids early CO 2 and temperature spikes, assuring that Earth was in energy balance. Between the LGM (19–21 kyBP) and Holocene (6.5–7.5 kyBP), GHG forcing increased 2.25 W/m 2 with 77% from CO 2 . Between the PGM and Eemian, GHG forcing increased 2.30 W/m 2 with 79% from CO 2 .
![abstract for global warming research paper Dome C temperature (Jouzel et al. [40]) and multi-ice core GHG amounts (Schilt et al. [47]). Green bars (1–5, 6.5–7.5, 18–21, 120–126, 137–144 kyBP) are periods of calculations.](https://oup.silverchair-cdn.com/oup/backfile/Content_public/Journal/oocc/3/1/10.1093_oxfclm_kgad008/1/m_kgad008f3.jpeg?Expires=1726813425&Signature=LvklB06gNIfNXDemtKM1ahXnMfPCJhvJJZufeiJt1poWC5mAYCrLayeLx8oTZF8t2JS1wAtwBRfMsxiWYHLfKnl9ccGaunZrEHr1AZQKlK2iopS2R~Zww0Ndf1OIWSvcU8BsRkAt8d~niwWnu15MOSxt39--dreNQ~23EAUb8KS-Ju5mp4TRMlM~-1UN-LserKzZYDL6YODYfYUCTw~2ol7W-3H2xe400QZn9G8OM6k3VYZIACFUy6ac4zgARGc2siyTvmvEPFxZVcH8P~-I0J8OLS2Ca8LEod8hzuLA~FiMq7Oavb519m9bSbl761qryr9GwT7uO8OtZGovdvlucQ__&Key-Pair-Id=APKAIE5G5CRDK6RD3PGA)
Dome C temperature (Jouzel et al . [ 40 ]) and multi-ice core GHG amounts (Schilt et al . [ 47 ]). Green bars (1–5, 6.5–7.5, 18–21, 120–126, 137–144 kyBP) are periods of calculations.
Glacial-interglacial aerosol changes are not included as a forcing. Natural aerosol changes, like clouds, are fast feedbacks. Indeed, aerosols and clouds form a continuum and distinction is arbitrary as humidity approaches 100%. There are many aerosol types, including VOCs (volatile organic compounds) produced by trees, sea salt produced by wind and waves, black and organic carbon produced by forest and grass fires, dust produced by wind and drought, and marine biologic dimethyl sulfide and its secondary aerosol products, all varying geographically and in response to climate change. We do not know, or need to know, natural aerosol properties in prior eras because their changes are feedbacks included in the climate response. However, human-made aerosols are a climate forcing (an imposed perturbation of Earth’s energy balance). Humans may have begun to affect gases and aerosols in the latter Holocene (Aerosols section), but we minimize that issue by using the 6.5–7.5 kyBP window to evaluate climate sensitivity.
Earth’s surface change is the other forcing needed to evaluate ECS: (1) change of surface albedo (reflectivity) and topography by ice sheets, (2) vegetation change, e.g. boreal forests replaced by brighter tundra, and (3) continental shelves exposed by lower sea level. Forcing by all three can be evaluated at once with a GCM. Accuracy requires realistic clouds, which shield the surface. Clouds are the most uncertain feedback [ 52 ]. Evaluation is ideal for CMIP [ 53 ] (Coupled Model Intercomparison Project) collaboration with PMIP [ 54 ] (Paleoclimate Modelling Intercomparison Project); a study of LGM surface forcing could aid GCM development and assessment of climate sensitivity. Sherwood et al . [ 21 ] review studies of LGM ice sheet forcing and settle on 3.2 ± 0.7 W/m 2 , the same as IPCC AR4 [ 55 ]. However, some GCMs yield efficacies as low as ∼0.75 [ 56 ] or even ∼0.5 [ 57 ], likely due to cloud shielding. We found [ 7 ] a forcing of −0.9 W/m 2 for LGM vegetation by using the Koppen [ 58 ] scheme to relate vegetation to local climate, but we thought the model effect was exaggerated as real-world forests tends to shake off snow albedo effects. Kohler et al . [ 59 ] estimate a continental shelf forcing of −0.67 W/m 2 . Based on an earlier study [ 60 ] (hereafter Target CO 2 ), our estimate of LGM-Holocene surface forcing is 3.5 ± 1 W/m 2 . Thus, LGM (18–21 kyBP) cooling of 7°C relative to mid-Holocene (7 kyBP), GHG forcing of 2.25 W/m 2 , and surface forcing of 3.5 W/m 2 yield an initial ECS estimate 7/(2.25 + 3.5) = 1.22°C per W/m 2 . We discuss uncertainties in Equilibrium climate sensitivity section.
PGM-Eemian global warming provides a second assessment of ECS, one that avoids concern about human influence. PGM-Eemian GHG forcing is 2.3 W/m 2 . We estimate surface albedo forcing as 0.3 W/m 2 less than in the LGM because sea level was about 10 m higher during the PGM [ 61 ]. North American and Eurasian ice sheet sizes differed between the LGM and PGM [ 62 ], but division of mass between them has little effect on the net forcing ( Supplementary Fig. S4 [ 60 ]). Thus, our central estimate of PGM-Eemian forcing is 5.5 W/m 2 . Eemian temperature reached about +1°C warmer than the Holocene [ 63 ], based on Eemian SSTs of +0.5 ± 0.3°C relative to 1870–1889 [ 64 ], or +0.65 ± 0.3°C SST and +1°C global (land plus ocean) relative to 1880–1920. However, the PGM was probably warmer than the LGM; it was warmer at Dome C ( Fig. 2 ), but cooler at Dronning Maud Land [ 65 ]. Based on deep ocean temperatures (Cenozoic Era section), we estimate PGM-Eemian warming as 0.5°C greater than LGM-Holocene warming, that is 7.5°C. The resulting ECS is 7.5/5.5 = 1.36°C per W/m 2 . Although PGM temperature lacks quantification comparable to that of Seltzer et al . [ 51 ] and Tierney et al . [ 49 ] for the LGM, the PGM-Eemian warming provides support for the high ECS inferred from LGM-Holocene warming.
We conclude that ECS for climate in the Holocene-LGM range is 1.2°C ± 0.3°C per W/m 2 , where the uncertainty is the 95% confidence range. The uncertainty estimate is inherently subjective, as it depends mainly on the ice age surface albedo forcing. The GHG forcing and glacial-interglacial temperature change are well-defined, but the efficacy of ice age surface forcing varies among GCMs. This variability is likely related to cloud shielding of surface albedo, which reaffirms the need for a focus on precise cloud observations and modeling.
State dependence of climate sensitivity
ECS based on glacial-interglacial climate is an average for global temperatures −7°C to +1°C relative to the Holocene and in general differs for other climate states because water vapor, aerosol-cloud and sea ice feedbacks depend on the initial climate. However, ECS is rather flat between today’s climate and warmer climate, based on a study [ 66 ] covering a range of 15 CO 2 doublings using an efficient GCM developed by Gary Russell [ 67 ]. Toward colder climate, ice-snow albedo feedback increases nonlinearly, reaching snowball Earth conditions—with snow and ice on land reaching sea level in the tropics—when CO 2 declines to a quarter to an eighth of its 1950 abundance ( Fig. 7 of the study) [ 66 ]. Snowball Earth occurred several times in Earth’s history, most recently about 600 million years ago [ 68 ] when the Sun was 6% dimmer [ 69 ] than today, a forcing of about –12 W/m 2 . Toward warmer climate, the water vapor feedback increases as the tropopause rises [ 70 ], the tropopause cold trap disappearing at 32 × CO 2 ( Fig. 7 ) [ 66 ]. However, for the range of ECS of practical interest—say from half preindustrial CO 2 to 4 × CO 2 —state dependence of ECS is small compared to state dependence of ESS.
Earth system sensitivity (ESS) includes amplifying feedbacks of GHGs and ice sheets [ 71 ]. When we consider CO 2 change as a known forcing, other GHGs provide a feedback that is smaller than the ice sheet feedback, but not negligible. Ice core data on GHG amounts show that non-CO 2 GHGs—including O 3 and stratospheric H 2 O produced by changing CH 4 —provide about 20% of the total GHG forcing, not only on average for the full glacial-interglacial change, but as a function of global temperature right up to +1°C global temperature relative to the Holocene ( Supplementary Fig. S5 ). Atmospheric chemistry modeling suggests that non-CO 2 GHG amplification of CO 2 forcing by about a quarter continues into warmer climate states [ 72 ]. Thus, for climate change in the Cenozoic era, we approximate non-CO 2 GHG forcing by increasing the CO 2 forcing by one-quarter.
Ice sheet feedback, in contrast to non-CO 2 GHG feedback, is highly nonlinear. Preindustrial climate was at most a few halvings of CO 2 from runaway snowball Earth and LGM climate was even closer to that climate state. The ice sheet feedback is reduced as Earth heads toward warmer climate today because already two-thirds of LGM ice has been lost. Yet remaining ice on Antarctica and Greenland constitutes a powerful feedback, which humanity is about to bring into play. We can illuminate that feedback and the climate path Earth is now on by examining data on the Cenozoic era—which includes CO 2 levels comparable to today’s amount—but first we must consider climate response time.
In this section we define response functions for global temperature and Earth’s energy imbalance that help reveal the physics of climate change. Cloud feedbacks amplify climate sensitivity and thus increase eventual heat uptake by the ocean, but cloud feedbacks also have the potential to buffer the rate at which the ocean takes up heat, thus increasing climate response time.
Climate response time was surprisingly long in our climate simulations [ 7 ] for the 1982 Ewing Symposium. The e-folding time—the time for surface temperature to reach 63% of its equilibrium response—was about a century. The only published atmosphere-ocean GCM—that of Bryan and Manabe [ 73 ]—had a response time of 25 years, while several simplified climate models referenced in our Ewing paper had even faster responses. The longer response time of our climate model was largely a result of high climate sensitivity—our model had an ECS of 4°C for 2 × CO 2 while the Bryan and Manabe model had an ECS of 2°C.
The physics is straightforward. If the delay were a result of a fixed source of thermal inertia, say the ocean’s well-mixed upper layer, response time would increase linearly with ECS because most climate feedbacks come into play in response to temperature change driven by the adjusted forcing, not in direct response to the forcing. Thus, a model with ECS of 4°C takes twice as long to reach full response as a model with ECS of 2°C, if the mixed layer provides the only heat capacity. However, while the mixed layer is warming, there is exchange of water with the deeper ocean, which slows the mixed layer warming. The longer response time with high ECS allows more of the ocean to come into play. If mixing into the deeper ocean is approximated as diffusive, surface temperature response time is proportional to the square of climate sensitivity [ 74 ].
Slow climate response accentuates need for the ‘anticipation’ that E.E. David, Jr spoke about. If ECS is 4.8°C (1.2°C per W/m 2 ), more warming is in the pipeline than widely assumed. GHG forcing today already exceeds 4 W/m 2 . Aerosols reduce the net forcing to about 3 W/m 2 , based on IPCC estimates (Aerosols section), but warming still in the pipeline for 3 W/m 2 forcing is 2.4°C, exceeding warming realized to date (1.2°C). Slow feedbacks increase the equilibrium response even further (Summary section). Large warmings can be avoided via a reasoned policy response, but definition of effective policies will be aided by an understanding of climate response time.
Temperature response function
T G is the Green’s function estimate of global temperature at time t, λ (°C per W/m 2 ) the model’s equilibrium sensitivity, R the dimensionless temperature response function (% of equilibrium response), and dF e the forcing change per unit time, dt. Integration over time begins when Earth is in near energy balance, e.g. in preindustrial time. The response function yields an accurate estimate of global temperature change for a forcing that does not cause reorganization of ocean circulation. Accuracy of this approximation for temperature for one climate model is shown in Chart 15 in the Bjerknes presentation and wider applicability has been demonstrated [ 76 ].
We study ocean mixing effects by comparing two GCMs: GISS (2014) [ 77 ] and GISS (2020) [ 33 ], both models 6 described by Kelley et al . [ 32 ].Ocean mixing is improved in GISS (2020) by use of a high-order advection scheme [ 78 ], finer upper-ocean vertical resolution (40 layers), updated mesoscale eddy parameterization, and correction of errors in the ocean modeling code [ 32 ]. The GISS (2020) model has improved variability, including the Madden-Julian Oscillation (MJO), El Nino Southern Oscillation (ENSO) and Pacific Decadal Oscillation (PDO), but the spectrum of ENSO-like variability is unrealistic and its amplitude is excessive, as shown by the magnitude of oscillations in Fig. 4a . Ocean mixing in GISS (2020) may still be excessive in the North Atlantic, where the model’s simulated penetration of CFCs is greater than observed [ 79 ].

( a ) Global mean surface temperature response to instant CO 2 doubling and ( b ) normalized response function (percent of final change). Thick lines in Figs 4 and 5 are smoothed (yr1 no smoothing; yr2 3-yr mean; yr3–12 5-yr mean, yr13–300 25-yr mean; yr301–5000 101-yr mean).
Despite reduced ocean mixing, the GISS (2020) model surface temperature response is no faster than in the GISS (2014) model ( Fig. 4b ): it takes 100 years to reach within 1/e of the equilibrium response. Slow response is partly explained by the larger ECS of the GISS (2020) model, which is 3.5°C versus 2.7°C for the GISS (2014) model, but something more is going on in the newer model, as exposed by the response function of Earth’s energy imbalance.
Earth’s energy imbalance (EEI)
When a forcing perturbs Earth’s energy balance, the imbalance drives warming or cooling to restore balance. Observed EEI is now of order +1 W/m 2 (more energy coming in than going out) [ 80 ]. High accuracy of EEI is obtained by tracking ocean warming—the main repository for excess energy—and adding heat stored in warming continents and heat used in net ice melt [ 80 ]. Heat storage in air adds a small amount. Radiation balance measured from Earth-orbiting satellites cannot by itself define the absolute imbalance, but, when anchored to an in situ EEI value for a sufficient interval (e.g. 10 years), satellite Earth radiation budget observations [ 81 ] provide invaluable EEI data on finer temporal and spatial scales than the in situ data.
After a step-function forcing is imposed, EEI and global surface temperature must each approach a new equilibrium, but EEI does so more rapidly, especially for the GISS (2020) model ( Fig. 5 ). EEI in GISS (2020) needs only a decade to reach within 1/e of full response ( Fig. 5b ), but global surface temperature requires a century ( Fig. 4b ). Rapid decline of EEI—to half the forcing in 5 years ( Fig. 5a )—has practical implications. First, EEI defines the rate heat is pumped into the ocean, so if EEI is reduced, ocean warming is slowed. Second, rapid EEI decline implies that it is wrong to assume that global warming can be stopped by a reduction of climate forcing by the amount of EEI. Instead, the required reduction of forcing is larger than EEI. The difficulty in finding additional reduction in climate forcing of even a few tenths of a W/m 2 is substantial [ 63 ]. Calculations that help quantify this matter are discussed in Supplementary Material section SM8 .

( a ) Earth’s energy imbalance (EEI) for 2 × CO 2 , and ( b ) EEI normalized response function.
What is the physics behind the fast response of EEI? The 2 × CO 2 forcing and initial EEI are both nominally 4 W/m 2 . In the GISS (2014) model, the decline of EEI averaged over the first year is 0.5 W/m 2 ( Fig. 5a ), a moderate decline that might be largely caused by warming continents and thus increased heat radiation to space. In contrast, EEI declines 1.3 W/m 2 in the GISS (2020) model ( Fig. 5a ). Such a huge, immediate decline of EEI implies existence of an ultrafast climate feedback. Climate feedbacks are the heart of climate change and warrant discussion.
Slow, fast and ultrafast feedbacks
Charney et al . [ 4 ] described climate feedbacks without discussing time scales. At the 1982 Ewing Symposium, water vapor, clouds and sea ice were described as ‘fast’ feedbacks [ 7 ] presumed to change promptly in response to global temperature change, as opposed to ‘slow’ feedbacks or specified boundary conditions such as ice sheet size, vegetation cover, and atmospheric CO 2 amount, although it was noted that some specified boundary conditions, e.g. vegetation, in reality may be capable of relatively rapid change [ 7 ].
The immediate EEI response ( Fig. 5a ) implies a third feedback time scale: ultrafast. Ultrafast feedbacks are not a new concept. When CO 2 is doubled, the added infrared opacity causes the stratosphere to cool. Instant EEI upon CO 2 doubling is only F i = +2.5 W/m 2 , but stratospheric cooling quickly increases EEI to +4 W/m 2 [ 82 ]. All models calculate a similar radiative effect, so it is useful to define an adjusted forcing, F a , which is superior to F i as a measure of climate forcing. In contrast, if cloud change—the likely cause of the present ultrafast change—is lumped into the adjusted forcing, each climate model has its own forcing, losing the merit of a common forcing.
Kamae et al . [ 83 ] review rapid cloud adjustment distinct from surface temperature-mediated change. Clouds respond to radiative forcing, e.g. via effects on cloud particle phase, cloud cover, cloud albedo and precipitation [ 84 ]. The GISS (2020) model alters glaciation in stratiform mixed-phase clouds, which increases supercooled water in stratus clouds, especially over the Southern Ocean [ Fig. 1 in the GCM description [ 32 ]]. The portion of supercooled cloud water drops goes from too little in GISS (2014) to too much in GISS (2020). Neither model simulates well stratocumulus clouds, yet the models help expose real-world physics that affects climate sensitivity and climate response time. Several models in CMIP6 comparisons find high ECS [ 84 ]. For the sake of revealing the physics, it would be useful if the models defined their temperature and EEI response functions. Model runs of even a decade can define the important part of Figs 4a and 5a . Many short (e.g. 2-year) 2 × CO 2 climate simulations with each run beginning at a different point in the model’s control run, can define cloud changes to an arbitrary accuracy.
In this section, we use ocean sediment core data to explore climate change in the past 66 million years. This allows us to study warmer climates that are relevant to human-made climate forcing.
High equilibrium climate sensitivity that we have inferred, ECS = 1.2°C ± 0.3°C per W/m 2 , may affect interpretation of warmer climates. GCMs have difficulty in producing Pliocene warmth [ 85 ], especially in the Arctic, without large—probably unrealistic—CO 2 amounts. In addition, a coupled GCM/ice sheet model needs 700–840 ppm CO 2 for transition between glaciated and unglaciated Antarctica [ 86 ]. Understanding of these climate states is hampered by uncertainty in the forcings that maintained the climate, as proxy measures of CO 2 have large uncertainty.
Theory informs us that CO 2 is the principal control knob on global temperature [ 87 ]. Climate of the past 800 000 years demonstrates ( Fig. 2 ) the tight control. Our aim here is to extract Cenozoic surface temperature history from the deep ocean oxygen isotope δ 18 O and infer Cenozoic CO 2 history. Oxygen isotope data has high temporal resolution for the entire Cenozoic, which aids understanding of Cenozoic climate change and resulting implications for future climate. Our CO 2 analysis is a complement to proxy CO 2 measurements. Despite progress in estimating CO 2 via carbon isotopes in alkenones and boron isotopes in planktic foraminifera [ 88 ], there is wide scatter among results and fossil plant stomata suggest smaller CO 2 amounts [ 89 ].
Deep ocean temperature and sea level from δ 18 O
![abstract for global warming research paper Global deep ocean δ18O. Black line: Westerhold et al. [90] data in 5 kyr bins until 34 MyBP and subsequently 2 kyr bins. Green line: Zachos et al. [44] data at 1 Myr resolution. Lower left: velocity [91] of Indian tectonic plate. PETM = Paleocene Eocene Thermal Maximum; EECO = Early Eocene Climatic Optimum; Oi-1 marks the transition to glaciated Antarctica; MCO = Miocene Climatic Optimum; NAIP = North Atlantic Igneous Province.](https://oup.silverchair-cdn.com/oup/backfile/Content_public/Journal/oocc/3/1/10.1093_oxfclm_kgad008/1/m_kgad008f6.jpeg?Expires=1726813425&Signature=3mdAM05VLstWdKVBE-1PkhNZ~Ryl6nt9V12tqxHdNw1slgfqfYXYOYnrBvXh~0ISAnSEvgGWZU6NTHKgvS9l6qCuQ-fuQM0oi4MdKTEi3339-j5ly~CgO~tCgDPkCbpqMUknh1TeCYJlbcxS8TrqCcMy9gLT~ZD~ToP9qH7p~n0yCit0XXlTQVDJTtpLyiclNdmXjpP8oGGnazf8loC6pyLngj6RuUPF3JZ4nzzLZ5k0k116~9AozD-a853DYLlVxyjvF1jaeD6eP1pr0BCHNOXCZEWjQl0HRd3zu2DpUKoOnSGO7T2BsGqqC398Rkff5lkK~2HqlfpkORBAVzj3kA__&Key-Pair-Id=APKAIE5G5CRDK6RD3PGA)
Global deep ocean δ 18 O. Black line: Westerhold et al . [ 90 ] data in 5 kyr bins until 34 MyBP and subsequently 2 kyr bins. Green line: Zachos et al . [ 44 ] data at 1 Myr resolution. Lower left: velocity [ 91 ] of Indian tectonic plate. PETM = Paleocene Eocene Thermal Maximum; EECO = Early Eocene Climatic Optimum; Oi-1 marks the transition to glaciated Antarctica; MCO = Miocene Climatic Optimum; NAIP = North Atlantic Igneous Province.
This equation is used for the early Cenozoic, up to the large-scale glaciation of Antarctica at ∼34 MyBP (Oi-1in Fig. 6 ). At larger δ 18 O (colder climate), lighter 16 O evaporates preferentially from the ocean and accumulates in ice sheets. In Zachos data, δ 18 O increases by 3 between Oi-1 and the LGM. Half of this δ 18 O change is due to the 6°C change of deep ocean temperature between Oi-1 (5°C) and the LGM (–1°C) [ 92 ]. The other 1.5 of δ 18 O change is presumed to be due to the ∼180 m sea level (SL) change between ice-free Earth and the LGM, with ∼60 m from Antarctic ice and 120 m from Northern Hemisphere ice. Thus, as an approximation to extract both SL and T do from δ 18 O, Hansen et al . [ 66 ] assumed that SL rose linearly by 60 m as δ 18 O increased from 1.75 to 3.25 and linearly by 120 m as δ 18 O increased from 3.25 to 4.75.
Zachos and Westerhold δ 18 O, SL and T do for the full Cenozoic, Pleistocene, and the past 800 000 years are graphed in Supplementary Material and sea level is compared to data of Rohling et al . [ 94 ]. We focus on the finer resolution W data. Differences between the W and Z data and interpretation of those differences are discussed in Paleocene Eocene Thermal Maximum section.
Cenozoic T S
In this section we combine the rich detail in T do provided by benthic δ 18 O with constraints on the range of Cenozoic T S from surface proxies to produce an estimated history of Cenozoic T S .
We expect T do change, which derives from sea surface temperature (SST) at high latitudes where deepwater forms, to approximate T S change when T do is not near the freezing point. Global SST change understates global T S (land plus ocean) change because land temperature response to a forcing exceeds SST response [ 95 ], e.g. the equilibrium global SST response of the GISS (2020) GCM to 2 × CO 2 is 70.6% of the global (land plus ocean) response. However, polar amplification of the SST response tends to compensate for SST undershoot of global T S change. Compensation is nearly exact at latitudes of North Atlantic deepwater formation for 2 × CO 2 climate change in the GISS (2020) climate model ( Fig. 7a ), but Southern Hemisphere polar amplification does not fully cover the 60–75°S latitudes where Antarctic bottom water forms.

( a ) Ratio of ΔSST (latitude) to global T S change for all ocean and the Atlantic Ocean, based on equilibrium response (years 4001–4500) in 2 × CO 2 simulations of GISS (2020) model. ( b ) ΔT, the amount by which T S change exceeds T do change, based on an exponential fit to the two data points provided by the Holocene and LGM (see text).
![abstract for global warming research paper Cenozoic temperature based on linear (Equations 15 and 16) and nonlinear (Equation 17) analyses. Antarctic Dome C data [40] (red) relative to last 1000 years are multiplied by 0.6 to account for polar amplification and 14°C is added for absolute scale.](https://oup.silverchair-cdn.com/oup/backfile/Content_public/Journal/oocc/3/1/10.1093_oxfclm_kgad008/1/m_kgad008f8.jpeg?Expires=1726813425&Signature=afU0Gl0Hzh~wYSKWE-gb4Ux1H27mzBdmTo0~VbKsbkNr8u3jVoqoLf~6ZXtl0neJGcDIHVpUj104Tl8K2~4ooHiU2fM~HHXADNvNFzmPZ3RHfsXMwSZGfsDJExtUznqLFKDSWLM3hdU0KDO00COpQ4-GVO3GvEO2RC~njnTcAuH19HWvEZklFbT1pqhbGlT8fg5Q0TDfnjFhUzpee~6UOKGXoXkJWkQMAgTAp59sYsPACab746v9X-kmAesL-AthawakUkc0vA1ipjdCY2wgOhHUbGhlfXI4oFDTVVbVCLATrBcKKHTyNWbcmopVGq69KV~pO51nczJuVGbmxq26NQ__&Key-Pair-Id=APKAIE5G5CRDK6RD3PGA)
Cenozoic temperature based on linear ( Equations 15 and 16 ) and nonlinear ( Equation 17 ) analyses. Antarctic Dome C data [ 40 ] (red) relative to last 1000 years are multiplied by 0.6 to account for polar amplification and 14°C is added for absolute scale.
The result is a consistent analysis of global T S for the entire Cenozoic ( Fig. 8b ). Oxygen isotope δ 18 O of deep ocean foraminifera reproduces glacial-interglacial temperature change well; more detailed agreement is not expected as Antarctic ice core data are for a location that moves, especially in altitude. Our interest is in warmer global climate and its relevance to upcoming human-caused climate change. For that purpose, we want to know the forcing that drove Cenozoic climate change. With the assumption that non-CO 2 GHG forcings provide 20% of the total GHG forcing, it is not difficult to infer the CO 2 abundance required to cause the Cenozoic temperature history in Fig. 8b . Considering the large disagreement among proxy CO 2 measures, this indirect measure of CO 2 via global T S may provide the most accurate Cenozoic CO 2 history.
Cenozoic CO 2
All quantities are known except ΔF CO2 (t), which is thus defined. Cenozoic CO 2 (t) for specified ECS is obtained from T S (t) using the CO 2 radiative forcing equation ( Table 1 , Supplementary Material ). Resulting CO 2 ( Fig. 9 ) is about 1,200 ppm at the EECO, 450 ppm at Oi-1, and 325 ppm in the Pliocene for ECS = 1.2°C per W/m 2 . For ECS = 1°C—about as low as we believe plausible—Pliocene CO 2 is near 350 ppm, rising only to ∼500 ppm at Oi-1 and ∼1500 ppm at EECO.
![abstract for global warming research paper Cenozoic CO2 estimated from δ18O of Westerhold et al. (see text). Black lines are for ECS = 1.2°C per W/m2; red and green curves (ECS = 1.0 and 1.4°C per W/m2) are 1 My smoothed. Blue curves (last 800 000 years) are Antarctica ice core data [41].](https://oup.silverchair-cdn.com/oup/backfile/Content_public/Journal/oocc/3/1/10.1093_oxfclm_kgad008/1/m_kgad008f9.jpeg?Expires=1726813425&Signature=1h79g2V9Z1BI4D3euRmTMG1-vTH1Da7nTVshRpMYnyQNWNGIR59GhjFco5g5f~FGNU6d6tT3RShrzlIiM5lKRDeCzrvJb8SxrGIf~x88Nyw9h~iPfTpOlrmRblHTUbuZ3-dbhNmmv2ACCA9FhdPYiTbYNG9aheH7Izn7utfL0OMzPIh3isVvYxA~a~Rju3h3Npsw3cOLYVujj~1bE7HWw48-K7l-~hqXrgqoc~OgbxUGG77KjylTjHOhbMbFxb1dXy-NRmAd-TFXTPIXiLw2Fc7lPA88pcQN2dj~LoCmnEeA-6fWHAv-lpvn~QRo1~34wkQDk~eDS6dSNcWkM~XawA__&Key-Pair-Id=APKAIE5G5CRDK6RD3PGA)
Cenozoic CO 2 estimated from δ 18 O of Westerhold et al . (see text). Black lines are for ECS = 1.2°C per W/m 2 ; red and green curves (ECS = 1.0 and 1.4°C per W/m 2 ) are 1 My smoothed. Blue curves (last 800 000 years) are Antarctica ice core data [ 41 ].
Assumed Holocene CO 2 amount is also a minor factor. We tested two cases: 260 and 278 ppm ( Fig. 9 ). These were implemented as the CO 2 values at 7 kyBP, but Holocene-mean values are similar—a few ppm less than CO 2 at 7 kyBP. Holocene = 278 ppm increases CO 2 about 20 ppm between today and Oi-1, and about 50 ppm at the EECO. However, Holocene CO 2 278 ppm causes the amplitude of inferred glacial-interglacial CO 2 oscillations to be less than reality ( Fig. 9b ), providing support for the Holocene 260 ppm level and for the interpretation that high late-Holocene CO 2 was due to human influence. Proxy measures of Cenozoic CO 2 yield a notoriously large range. A recent review [ 88 ] constructs a CO 2 history with Loess-smoothed CO 2 ∼700–1100 ppm at Oi-1. That high Oi-1 CO 2 amount is not plausible without overthrowing the concept that global temperature is a response to climate forcings. More generally, we conclude that actual CO 2 during the Cenozoic was near the low end of the range of proxy measurements.
Interpretation of Cenozoic T S and CO 2
In this section we consider Cenozoic T S and CO 2 histories, which are rich in insights about climate change with implications for future climate.
In Target CO 2 [ 60 ] and elsewhere [ 98 ] we argue that the broad sweep of Cenozoic temperature is a result of plate tectonic (popularly ‘continental drift’) effects on CO 2 . Solid Earth sources and sinks of CO 2 are not balanced at any given time. CO 2 is removed from surface reservoirs by: (1) chemical weathering of rocks with deposition of carbonates on the ocean floor, and (2) burial of organic matter [ 99 , 100 ]. CO 2 returns via metamorphism and volcanic outgassing at locations where oceanic crust is subducted beneath moving continental plates. The interpretation in Target CO 2 was that the main Cenozoic source of CO 2 was associated with the Indian plate ( Fig. 10 ), which separated from Pangea in the Cretaceous [ 101 , 102 ] and moved through the Tethys (now Indian) Ocean at a rate exceeding 10 cm/year until collision with the Eurasian plate at circa 50 MyBP. Associated CO 2 emissions include those from formation of the Deccan Traps 7 in western India (a large igneous province, LIP, formed by repeated deposition of large-scale flood basalts), the smaller Rajahmundry Traps [ 103 ] in eastern India, and metamorphism and vulcanism associated with the moving Indian plate. The Indian plate slowed circa 60 Mya (inset, Fig. 6 ) before resuming high speed [ 91 ], leaving an indelible signature in the Cenozoic δ 18 O history ( Fig. 6 ) that supports our interpretation of the CO 2 source. Since the continental collision, subduction and CO 2 emissions continue at a diminishing rate as the India plate underthrusts the Asian continent and pushes up the Himalayan mountains [ 104 ]. We interpret the decline of CO 2 over the past 50 million years as, at least in part, a decline of the metamorphic source from continued subduction of the Indian plate, but burial of organic matter and increased weathering due to exposure of fresh rock by Himalayan uplift [ 105 ] may contribute to CO 2 drawdown. Quantitative understanding of these processes is limited [ 106 ], e.g. weathering is both a source and sink of CO 2 [ 107 ].
![abstract for global warming research paper Continental configuration 56 MyBP [97]. Continental shelves (light blue) were underwater as little water was locked in ice. The Indian plate was moving north at about 15 cm per year.](https://oup.silverchair-cdn.com/oup/backfile/Content_public/Journal/oocc/3/1/10.1093_oxfclm_kgad008/1/m_kgad008f10.jpeg?Expires=1726813425&Signature=JQXo1K1qJWicP0TFTU566a9U~xD8ZvsNnZvXvXWZ0JZJs~969fpUKA5OrVUwALVnCeWyETrFR93y3PRJeAPEn5sQogxW3AcW4ajof6C1~urFoi~WMPYCbvNxTEVKhtpJyVxHmXOXHQI9AxUtTItdKycFohtPd4IvPGYaB7jrXVhy4rp1GNkqmj3fKKWtRLW~rY0mrXtFKRrN01eeTUC-GrWLcNhVOMybTTHdP2Tex2Zs8Y66Y0t4W~PdIR5oZ26f-hOZTJgZ7SGq3eVhURrc7nZ1cvPcbXULAZcHx9ynV2HTNLG6ybw~5iQK140BNlmVRr-V9GfRs~Wqcf5orjODAQ__&Key-Pair-Id=APKAIE5G5CRDK6RD3PGA)
Continental configuration 56 MyBP [ 97 ]. Continental shelves (light blue) were underwater as little water was locked in ice. The Indian plate was moving north at about 15 cm per year.
This picture for the broad sweep of Cenozoic CO 2 is consistent with current understanding of the long-term carbon cycle [ 108 ], but relative contributions of metamorphism [ 106 ] and volcanism [ 109 ] are uncertain. Also, emissions from rift-induced Large Igneous Provinces (LIPs) [ 110 , 111 ] contribute to long-term change of atmospheric CO 2 , with two cases prominent in Fig. 6 . The Columbia River Flood Basalt at ca. 17–15 MyBP was a principal cause of the Miocene Climatic Optimum [ 112 ], but the processes are poorly understood [ 113 ]. A more dramatic event occurred as Greenland separated from Europe, causing a rift in the sea floor; flood basalt covered more than a million square kilometers with magma volume 6–7 million cubic kilometers [ 111 ]—the North Atlantic Igneous Province (NAIP). Flood basalt volcanism occurred during 60.5–54.5 MyBP, but at 56.1 ± 0.5 MyBP melt production increased by more than a factor of 10, continued at a high level for about a million years, and then subsided ( Fig. 5 of Storey et al . [ 114 ]). The striking Paleocene-Eocene Thermal Maximum (PETM) δ 18 O spike ( Fig. 6 ) occurs early in this million-year bump-up of δ 18 O. Svensen et al . [ 115 ] proposed that the PETM was initiated by the massive flood basalt into carbon-rich sedimentary strata. Gutjahr et al . [ 116 ] developed an isotope analysis, concluding that most of PETM carbon emissions were volcanic, with climate-driven carbon feedbacks playing a lesser role. Yet other evidence [ 117 ], while consistent with volcanism as a trigger for the PETM, suggests that climate feedback—perhaps methane hydrate and peat CO 2 release—may have caused more than half of the PETM warming. Berndt et al . [ 118 ] describe extensive shallow-water vents that likely released CH 4 as well as CO 2 during the NAIP activity. We discuss PETM warming and CO 2 levels below, but first we must quantify the mechanisms that drove Cenozoic climate change and consider where Earth’s climate was headed before humanity intervened.
The sum of climate forcings (CO 2 and solar) and slow feedbacks (ice sheets and non-CO 2 GHGs) that maintained EECO warmth was 12.5 W/m 2 ( Fig. 11 ). CO 2 forcing of 9.1 W/m 2 combined with solar forcing of—1.2 W/m 2 to yield a total forcing 8 8 W/m 2 . Slow feedbacks were 4.5 W/m 2 forcing (ice albedo = 2 W/m 2 and non-CO 2 GHGs = 2.5 W/m 2 ). With today’s solar irradiance, human-made GHG forcing required for Earth to return to EECO warmth is 8 W/m 2 . Present human-made GHG forcing is 4.6 W/m 2 relative to 7 kyBP. 9 Equilibrium response to this forcing includes the 2 W/m 2 ice sheet feedback and 25% amplification (of 6.6 W/m 2 ) by non-CO 2 GHGs, yielding a total forcing plus slow feedbacks of 8.25 W/m 2 . Thus, equilibrium global warming for today’s GHGs is 10°C. 10 If human-made aerosol forcing is −1.5 W/m 2 and remains at that level indefinitely, equilibrium warming for today’s atmosphere is reduced to 8°C. Either 10°C or 8°C dwarfs observed global warming of 1.2°C to date. Most of the equilibrium warming for today’s atmosphere has not yet occurred and need not occur (Earth’s energy imbalance section).

Climate forcings and slow feedbacks relative to 7 kyBP from terms in Equations (21–23) .
Prospects for another snowball Earth
We would be remiss if we did not comment on the precipitous decline of Earth’s temperature over the last several million years. Was Earth falling off the table into another Snowball Earth?
Global temperature plummeted in the past 50 million years, with growing, violent, oscillations ( Figs 6 and 7 ). Glacial-interglacial average CO 2 declined from about 325 ppm to 225 ppm in the past five million years in an accelerating decline ( Fig. 9a ). As CO 2 fell to 180 ppm during recent glacial maxima, an ice sheet covered most of Canada and reached midlatitudes in the U.S. Continents in the current supercontinent cycle [ 101 ] are now dispersed, with movement slowing to 2–3 cm/year. Emissions from the last high-speed high-impact tectonic event—collision of the Indian plate with Eurasia—are fizzling out. The most recent large igneous province (LIP) event—the Columbia River Flood Basalt about 15 million years ago ( Fig. 6 )—is no longer a factor, and there is no evidence of another impending LIP. Snowball conditions are possible, even though the Sun’s brightness is increasing and is now almost 6% greater [ 69 ] than it was at the last snowball Earth, almost 600 million years ago [ 68 ]. Runaway snowball likely requires only 1–2 halvings [ 66 ] of CO 2 from the LGM 180 ppm level, i.e. to 45–90 ppm. Although the weathering rate declines in colder climate [ 119 ], weathering and burial of organic matter continue, so decrease of atmospheric CO 2 could have continued over millions of years, if the source of CO 2 from metamorphism and vulcanism continued to decline.
Another factor that may have contributed to cooling in the Pliocene is uplift and poleward movement of Greenland that accelerated about 5 MyBP [ 120 ], which likely enhanced glaciation of Greenland and should be accounted for in simulations of Pliocene climate change. We conclude that, in the absence of human activity, Earth may have been headed for snowball Earth conditions within the next 10 or 20 million years, but the chance of future snowball Earth is now academic. Human-made GHG emissions remove that possibility on any time scale of practical interest. Instead, GHG emissions are now driving Earth toward much warmer climate.
Paleocene eocene thermal maximum (PETM)
The PETM event provides a benchmark for assessing the potential impact of the human-made climate forcing and the time scale for natural recovery of the climate system.
Westerhold [ 90 ] data have 10°C deep ocean warming at the PETM ( Figs 8 and 12a ), which exceeds proxy-derived surface warming. Low latitude SST data have 3–4°C PETM warming [ 121 ]. Tierney et al . [ 122 ] obtain PETM global surface warming 5.6°C (5.4–59°C, 95% confidence) via analysis of proxy surface temperature data that accounts for patterns of temperature change. Zachos [ 44 ] data have a deep ocean warming similar to the proxy-based surface warming. These warming estimates can be reconciled, but first let’s note the practical importance of the PETM.
Pre-PETM (56–56.4 MyBP) CO 2 is 910 ppm in our analysis for the most likely ECS (1.2°C per W/m 2 ). Peak PETM CO 2 required to yield the 5.6°C global surface warming estimate of Tierney et al . [ 122 ] is then 1630 ppm if CO 2 provides 80% of the GHG forcing, thus less than a doubling of CO 2 . (In the unlikely case that CO 2 caused 100% of the GHG forcing, required CO 2 is 1780, not quite a doubling.) CO 2 amounts for ECS = 1.0 and 1.4°C per W/m 2 are 1165 and 760 ppm in the pre-PETM and 2260 and 1270 ppm at peak PETM, respectively. In all these ECS cases, the CO 2 forcing of the PETM is less than or about a CO 2 doubling. Our assumed 20% contribution by non-CO 2 GHGs (amplification factor 1.25, Climate sensitivity (ECS and ESS) section), is nominal; Hopcroft et al ., e.g. estimate a 30% contribution from non-CO 2 GHGs [ 123 ], thus an amplification factor 1.43.
Thus, today’s human-made GHG forcing (4.6 W/m 2 , growing 0.5 W/m 2 per decade) is already at least comparable to the PETM forcing, although the net human-made forcing including aerosols has probably not reached the PETM forcing. However, there are two big differences between the PETM and today. First, there were no large ice sheets on Earth in the PETM era. Ice sheets on Antarctica and Greenland today make Earth system sensitivity (ESS) greater than it was during the PETM. Equilibrium response to today’s GHG climate forcing would include deglaciation of Antarctica and Greenland, sea level rise of 60 m (200 feet), and surface albedo forcing (slow feedback) of 2 W/m 2 . The second difference between the PETM and today is the rate of change of the climate forcing. Most of today’s climate forcing was introduced in a century, which is 10 times or more faster than the PETM forcing growth. Although a bolide impact [ 124 ] has been proposed as a trigger for the PETM, the issue is the time scale on which the climate forcing—increased GHGs—occurred. Despite uncertainty in the carbon source(s), data and modeling point to duration of a millennium or more for PETM emissions [ 121 , 125 ].
Better understanding of the PETM could inform us on climate feedbacks. Gutjahr et al . [ 116 ] argue persuasively that PETM emissions were mostly volcanic, yet we know of no other large igneous province that produced such great, temporally-isolated, emissions. Further, Cenozoic orbitally-driven hyperthermal events [ 126 ] testify to large CO 2 feedbacks. Northern peatlands today contain more than 1000 Gt carbon [ 127 ], much of which can be mobilized at PETM warming levels [ 128 ]. The double peak in deep ocean δ 18 O (thus in temperature, cf. Fig. 12 , where each square is a binning interval of 5000 years) is also found in terrestrial data [ 129 ]. Perhaps the sea floor rift occurred in two bursts, or the rift was followed tens of thousands of years later by methane hydrate release as a feedback to the ocean warming; much of today’s methane hydrate is in stratigraphic deposits hundreds of meters below the sea floor, where millennia may pass before a thermal wave from the surface reaches the deposits [ 130 ]. Feedback emissions, especially from permafrost, seem to be more chronic than catastrophic, but stabilization of climate may require cooling that terminates growth of those feedbacks (Summary section). The PETM provides perhaps the best empirical check on understanding of the atmospheric lifetime of fossil fuel CO 2 [ 131 ], but for that purpose we must untangle as well as possible the time dependence of the PETM CO 2 source and feedbacks. If continuing magma flow or a slow-release feedback is a substantial portion of PETM CO 2 , the CO 2 lifetime inferred from post-PETM CO 2 recovery may be an exaggeration.
![abstract for global warming research paper Temperature and CO2 implied by Westerhold et al. [90] δ18O, if surface warming equaled deep ocean warming. In reality, the unique PETM event had surface warming ∼5.6°C, which implies a peak PETM CO2 of about 1630 ppm (see text).](https://oup.silverchair-cdn.com/oup/backfile/Content_public/Journal/oocc/3/1/10.1093_oxfclm_kgad008/1/m_kgad008f12.jpeg?Expires=1726813425&Signature=hpvHZ1kWJe9I99KmYN84svaEwIwFvVT181XbsP4h~0CL7zFahf4Xg2B4LbWY4voo1KOnAWx8oPsu7IgKz-87gX5CT6S7Uq2-oVW-qc9A7yUvI~4ftKk-QA8jT0bco9nBcDFdZgStLWsMv0mWxc87c2IlPboiaVEqFXkUnsqXYmYUjK5k0HVKvXD84WVO0T06sNkJg-vQ-QSTwHhHFSh8KtLZKbcb7fGX3bCUPvsDrCc8VDGpo~l1xLOONDRyg4IvACmvFhTuVdoILIMJC9imnbJKe9M8bsDhoz4m9lbVC4WzqDxyFfMbOAr93D~7DVHZMBNShNKClOcgzefqynw30A__&Key-Pair-Id=APKAIE5G5CRDK6RD3PGA)
Temperature and CO 2 implied by Westerhold et al . [ 90 ] δ 18 O, if surface warming equaled deep ocean warming. In reality, the unique PETM event had surface warming ∼5.6°C, which implies a peak PETM CO 2 of about 1630 ppm (see text).
The PETM draws attention to differences between the Westerhold (W) and Zachos (Z) δ 18 O data. Zachos attributes the larger PETM response in W data to the shallow (less than 1 km) depth of the Walvis Ridge core in the Southeast Atlantic that anchors the PETM period in the W data (see Supplementary Material SM9 ). Given that the PETM was triggered by a rift in the floor of the North Atlantic and massive lava injection, it is not surprising that ocean temperature was elevated and circulation disrupted during the PETM. Nunes and Norris [ 132 ] conclude that ocean circulation changed at the start of the PETM with a shift in location of deep-water formation that delivered warmer waters to the deep sea, a circulation change that persisted at least 40 000 years. With regard to differences in the early Cenozoic, Zachos notes ( Supplementary Material SM9 ) a likely bias in the Z data with a heavy weighting of data from Southern Ocean sites (Kerguelen Plateau and Maud Rise), which were intended for study of climate of Antarctica and the Southern Ocean.
Differences between the W and Z data sets have limited effect on our paper, as we apply separate scaling ( Equations 7–14 ) to W and Z data to match observations at the LGM, mid-Holocene, and Oi-1 points. This approach addresses, e.g. the cumulative effect in combining data splices noted by Zachos in SM9. Further, we set the EECO global temperature relative to the Holocene and the PETM temperature relative to pre-PETM based on proxy-constrained, full-field, GCM analyses of Tierney et al . [ 122 ] and Zhu et al . [ 96 ] Nevertheless, there is much to learn from more precise study of the Cenozoic in general and the PETM in particular.
Policy implications require first an understanding of the role of aerosols in climate change.
The role of aerosols in climate change is uncertain because aerosol properties are not measured well enough to define their climate forcing. In this section we estimate aerosol climate forcing via aerosol effects on Earth’s temperature and Earth’s energy imbalance.
Aerosol impact is suggested by the gap between observed global warming and expected warming due to GHGs based on ECS inferred from paleoclimate ( Fig. 13 ). Expected warming is from Eq. 5 with the normalized response function of the GISS (2020) model. Our best estimate for ECS, 1.2°C per W/m 2 , yields a gap of 1.5°C between expected and actual warming in 2022. Aerosols are the likely cooling source. The other negative forcing discussed by IPCC—surface albedo change—is estimated by IPCC (Chapter 7, Table 7.8) to be –0.12 ± 0.1 W/m 2 , an order of magnitude smaller than aerosol forcing [ 12 ]. Thus, for clarity, we focus on GHGs and aerosols.
![abstract for global warming research paper Observed global surface temperature (black line) and expected GHG warming with two choices for ECS. The blue area is the estimated aerosol cooling effect. The temperature peak in the World War II era is in part an artifact of inhomogeneous ocean data in that period [63].](https://oup.silverchair-cdn.com/oup/backfile/Content_public/Journal/oocc/3/1/10.1093_oxfclm_kgad008/1/m_kgad008f13.jpeg?Expires=1726813425&Signature=UBT6xF~mWePnPibo6vc4Wfg4U5qDO5FRWQykhtGSoFCIExGaf88fXD001KgA8PRLOx-2lAYJf96sR0U0jsifr7AURQ3h5J5GsXtbq22EiovcRMT6eGSoQYVh7xaVLRS1yKTsJTCHPDrpexoXRn4jwcSzo9PKXK4yPNbn3W27SF8qYH2p-Y5XEM9Gg-kMxoaUqYqukkr5vzB3ES03RriaJGHTXf-tP5bPCnPXucfgMbtkl0kmsitNA58V5xcCY5SIIGyeqA4LdeJdrIUFnPs3yqb3bWBNbzXXzhqtR-roPd02d5uK39sfKwLIO8H1cZXtKZRJGZmxCgjabQPZmyHDbg__&Key-Pair-Id=APKAIE5G5CRDK6RD3PGA)
Observed global surface temperature (black line) and expected GHG warming with two choices for ECS. The blue area is the estimated aerosol cooling effect. The temperature peak in the World War II era is in part an artifact of inhomogeneous ocean data in that period [ 63 ].
Absence of global warming over the period 1850–1920 ( Supplementary Fig. S1 of IPCC AR6 WG1 report [ 12 ]) is a clue about aerosol forcing. GHG forcing increased 0.54 W/m 2 in 1850–1920, which causes expected warming 0.3–0.4°C by 1920 for ECS = 1.2°C per W/m 2 ( Equation 5 ). Natural forcings—solar irradiance and volcanoes—may contribute to lack of warming, but a persuasive case for the required forcing has not been made. Human-made aerosols are the likely offset of GHG warming. Such aerosol cooling is a Faustian bargain [ 98 ] because payment in enhanced global warming will come due once we can no longer tolerate the air pollution. Ambient air pollution causes millions of deaths per year, with particulates most responsible [ 133 , 134 ].
Evidence of aerosol forcing in the Holocene
In this section we infer evidence of human-made aerosols in the last half of the Holocene from the absence of global warming. Some proxy-based analyses [ 135 ] report cooling in the last half of the Holocene, but a recent analysis [ 50 ] that uses GCMs to overcome spatial and temporal biases in proxy data finds rising global temperature in the first half of the Holocene followed by nearly constant temperature in the last 6000 years until the last few centuries ( Fig. 14 ). Antarctic, deep ocean, and tropical sea surface data all show stable temperature in the last 6000 years ( Supplementary Fig. S6 of reference [ 60 ]). GHG forcing increased 0.5 W/m 2 during those 6000 years ( Fig. 15 ), yet Earth did not warm. Fast feedbacks alone should yield at least +0.5°C warming and 6000 years is long enough for slow feedbacks to also contribute. How can we interpret the absence of warming?
![abstract for global warming research paper Global mean surface temperature change over the past 24 ky, reproduced from Fig. 2 of Osman et al. [50] including Last Millennium reanalysis of Tardif et al. [136].](https://oup.silverchair-cdn.com/oup/backfile/Content_public/Journal/oocc/3/1/10.1093_oxfclm_kgad008/1/m_kgad008f14.jpeg?Expires=1726813425&Signature=5QHLyPIipwUDeYANqk2khtXOcViSY9OUXb9ViL7bvBFRZetWBBGnnkGqIb1AIwcI7gO2Jba6AC-mW~ENkdZrWSmYecqkydIAEvSqhiMkSsxR-p8bW3dJlPNwxO2FNaj46Y2MGRv3e9XCWg4wQMf6K2iJIky-ZGLLJSGJcmeVZWBmVCf3~JvTgX8nf~WWFIcHO5LeYT4HqUEf~HUhELCQduosgZirlwgIyQCK0fXdvmXttYXWJ86Xgb02Zs4WQFi64JGeZWTCQjJArVL-QrE0roF5LkoLVujwjPmsTNJ4Ip-yAMs3ykBrY9hjojsUJBGCc7EBVmRYjdM1nwL~U0w4vQ__&Key-Pair-Id=APKAIE5G5CRDK6RD3PGA)
Global mean surface temperature change over the past 24 ky, reproduced from Fig. 2 of Osman et al . [ 50 ] including Last Millennium reanalysis of Tardif et al . [ 136 ].
![abstract for global warming research paper GHG climate forcing in past 20 ky with vertical scale expanded for the past 10 ky on the right. GHG amounts are from Schilt et al. [47]. Formulae for forcing are in Supplementary Material.](https://oup.silverchair-cdn.com/oup/backfile/Content_public/Journal/oocc/3/1/10.1093_oxfclm_kgad008/1/m_kgad008f15.jpeg?Expires=1726813425&Signature=w82ap89cJag-iNoh1EMJaaKJPzseSz9UjPx-mvWaEl2~mZVgviYZaAsyTk9ay~2vKC3u-BZcdOBmM2AeQBgcTtqXKXFcjoNaF8Q245EbHYbBgc5ABS~VfpjkvnMo5sRE6h~d~cvvBqTII0~gR~4HYZvvucCFHgg9-1hk7xSaCpzNjtuHujvJfWPyYne8yurSPvd8ilTs4W~5LkJdKzJrHegcB1k25p2j0jB94~FUcMCHzuBw32jYSHs8eVwsBFob-Z1l8fqd3tuI4QNrPso-7Vi5WSH4~PbxUmLqJ4pKEgYUp1mxLbsMipNgO41SVSFmzdUqVL4~kczsXsgo77yoTg__&Key-Pair-Id=APKAIE5G5CRDK6RD3PGA)
GHG climate forcing in past 20 ky with vertical scale expanded for the past 10 ky on the right. GHG amounts are from Schilt et al . [ 47 ]. Formulae for forcing are in Supplementary Material .
Humanity’s growing footprint deserves scrutiny. Ruddiman’s suggestion that deforestation and agriculture began to affect CO 2 6500 year ago and rice agriculture began to affect CH 4 5000 years ago has been criticized [ 46 ] mainly because of the size of proposed sources. Ruddiman sought sources sufficient to offset declines of CO 2 and CH 4 in prior interglacial periods, but such large sources are not needed to account for Holocene GHG levels. Paleoclimate GHG decreases are slow feedbacks that occur in concert with global cooling. However, if global cooling did not occur in the past 6000 years, feedbacks did not occur. Earth orbital parameters 6000 years ago kept the Southern Ocean warm, as needed to maintain strong overturning ocean circulation [ 137 ] and minimize carbon sequestration in the deep ocean. Maximum insolation at 60°S was in late-spring (mid-November); since then, maximum insolation at 60°S slowly advanced through the year, recently reaching mid-summer (mid-January, Fig. 26b of Ice Melt [ 13 ]). Maximum insolation from late-spring through mid-summer is optimum to warm the Southern Ocean and promote early warm-season ice melt, which reduces surface albedo and magnifies regional warming [ 45 ].
GHG forcing of –0.2 W/m 2 in 10–6 kyBP ( Fig. 15 ) was exceeded by forcing of +1 W/m 2 due to ice sheet shrinkage ( Supplementary Material in Target CO 2 [ 60 ]) for a 40 m sea level rise ( Fig. 16 ). Net 0.8 W/m 2 forcing produced expected 1°C global warming ( Fig. 14 ). The mystery is the absence of warming in the past 6000 years. Hansen et al . [ 45 ] suggested that aerosol cooling offset GHG warming. Growing population, agriculture and land clearance produced aerosols and CO 2 ; wood was the main fuel for cooking and heating. Nonlinear aerosol forcing is largest in a pristine atmosphere, so it is unsurprising that aerosols tended to offset CO 2 warming as civilization developed. Hemispheric differences could provide a check. GHG forcing is global, while aerosol forcing is mainly in the Northern Hemisphere. Global offset implies a net negative Northern Hemisphere forcing and positive Southern Hemisphere forcing. Thus, data and modeling studies (including orbital effects) of regional response are warranted but beyond the scope of this paper.
![abstract for global warming research paper Sea level since the last glacial period relative to present. Credit: Robert Rohde [138].](https://oup.silverchair-cdn.com/oup/backfile/Content_public/Journal/oocc/3/1/10.1093_oxfclm_kgad008/1/m_kgad008f16.jpeg?Expires=1726813425&Signature=bcz7-hwHtvtSVGOdV7cKuwyTxYpOCD1nXKHlgtAgbCxcyCM6mTogjbDttY4aLbZgsLstK4yus2zsSIG-gXSE38KxC9Wg41B7DhXyYm9KnxAeQrhCPYg3OCZLwgGEps52WPPdzmthSL9o31qOAakRFdCYdgZUcVry-G663YOOFqJqTkvKEdIj6wYnewyn3Cl0duVQxDhJt7pUnv~LZchJWCsjKiHF66kG6iFU5FtILnwIuwy-fDlTp3H4LWNnC9M17sdmNRcMk~qAOJlKtdehpXsc5H5eUhezFpXhXXasxh-T~Mu0Zo~2sjHWSHW2X0VX7H1MfPy3ChajuDUcI5ReBw__&Key-Pair-Id=APKAIE5G5CRDK6RD3PGA)
Sea level since the last glacial period relative to present. Credit: Robert Rohde [ 138 ].
Industrial era aerosols
Scientific advances often face early resistance from other scientists [ 139 ]. Examples are the snowball Earth hypothesis [ 140 ] and the role of an asteroid impact in extinction of non-avian dinosaurs [ 141 ], which initially were highly controversial but are now more widely accepted. Ruddiman’s hypothesis, right or wrong, is still controversial. Thus, we minimize this issue by showing aerosol effects with and without preindustrial human-made aerosols.
Global aerosols are not monitored with detail needed to define aerosol climate forcing [ 142 , 143 ]. IPCC12 estimates forcing ( Fig. 17a ) from assumed precursor emissions, a herculean task due to many aerosol types and complex cloud effects. Aerosol forcing uncertainty is comparable to its estimated value ( Fig. 17a ), which is constrained more by observed global temperature change than by aerosol measurements [ 144 ]. IPCC’s best estimate of aerosol forcing ( Fig. 17 ) and GHG history define the percent of GHG forcing offset by aerosol cooling—the dark blue area in Fig. 17b . However, if human-made aerosol forcing was −0.5 W/m 2 by 1750, offsetting +0.5 W/m 2 GHG forcing, this forcing should be included. Such aerosol forcing—largely via effects of land use and biomass fuels on clouds—continues today. Thirty million people in the United States use wood for heating [ 145 ]. Such fuels are also common in Europe [ 146 , 147 ] and much of the world.

( a ) Estimated greenhouse gas and aerosol forcings relative to 1750 values. ( b ) Aerosol forcing as percent of GHG forcing. Forcings for dark blue area are relative to 1750. Light blue area adds 0.5 W/m 2 forcing estimated for human-caused aerosols from fires, biofuels and land use.
Figure 17b encapsulates two alternative views of aerosol history. IPCC aerosol forcing slowly becomes important relative to GHG forcing. In our view, civilization always produced aerosols as well as GHGs. As sea level stabilized, organized societies and population grew as coastal biologic productivity increased [ 148 ] and agriculture developed. Wood was the main fuel. Aerosols travel great distances, as shown by Asian aerosols in North America [ 149 ]. Humans contributed to both rising GHG and aerosol climate forcings in the past 6000 years. One result is that human-caused aerosol climate forcing is at least 0.5 W/m 2 more than usually assumed. Thus, the Faustian payment that will eventually come due is also larger, as discussed in Summary section.
Ambiguity in aerosol climate forcing
In this section we discuss uncertainty in the aerosol forcing. We discuss why global warming in the past century—often used to infer climate sensitivity—is ill-suited for that purpose.
Recent global warming does not yield a unique ECS because warming depends on three major unknowns with only two basic constraints. Unknowns are ECS, net climate forcing (aerosol forcing is unmeasured), and ocean mixing (many ocean models are too diffusive). Constraints are observed global temperature change and Earth’s energy imbalance (EEI) [ 80 ]. Knutti [ 150 ] and Hansen [ 75 ] suggest that many climate models compensate for excessive ocean mixing (which reduces surface warming) by using aerosol forcing less negative than the real world, thus achieving realistic surface warming. This issue is unresolved and complicated by the finding that cloud feedbacks can buffer ocean heat uptake (Climate response time section), affecting interpretation of EEI.
IPCC AR6 WG1 best estimate of aerosol forcing (Table AIII.3) [ 12 ] is near maximum (negative) value by 1975, then nearly constant until rising in the 21st century to –1.09 W/m 2 in 2019 ( Fig. 18 ). We use this IPCC aerosol forcing in climate simulations here. We also use an alternative aerosol scenario [ 151 ] that reaches –1.63 W/m 2 in 2010 relative to 1880 and –1.8 W/m 2 relative to 1850 ( Fig. 18 ) based on modeling of Koch [ 152 ] that included changing technology factors defined by Novakov [ 153 ]. This alternative scenario 11 is comparable to the forcing in some current aerosol models ( Fig. 18 ). Human-made aerosol forcing relative to several millennia ago may be even more negative, by about –0.5 W/m 2 as discussed above, but the additional forcing was offset by increasing GHGs and thus those additional forcings are neglected, with climate assumed to be in approximate equilibrium in 1850.
![abstract for global warming research paper Aerosol forcing relative to 1850 from IPCC AR6, an alternative aerosol scenario [151] two aerosol model scenarios of Bauer et al. [154].](https://oup.silverchair-cdn.com/oup/backfile/Content_public/Journal/oocc/3/1/10.1093_oxfclm_kgad008/1/m_kgad008f18.jpeg?Expires=1726813425&Signature=wsiIGe4FpmR~vifn8tmW~mh5EJ0OE~Wis-ohmK9dcT6MfcIT6OEUHNFc3HTaBumRFJyBf3UUjqA1Zft2YiDyKGcUBjCcI4-g-8RRViJ5UPxd-ZJIK6TIOBJhYaPExoKm4J5-yamhCMgPMwLAtv0N29V-3p22PLzSFhmbrzhb5Y~DkvEQTH-hkxfWtFPrYY9Z9v1LmUODe777accbN-rd9xnMSjUOLIGGSQQnL-Q8H24-Ji5U3mbRDLuhXPoFvtFgJAhFt3TEzd4SAZWAkkvHXnpcluQbwpYOl9twCLRiIpQFBaFGYETC6~z2ogVBFycweY~YcbCrDtnwX5wlmI8D1w__&Key-Pair-Id=APKAIE5G5CRDK6RD3PGA)
Aerosol forcing relative to 1850 from IPCC AR6, an alternative aerosol scenario [ 151 ] two aerosol model scenarios of Bauer et al . [ 154 ].
Many combinations of climate sensitivity and aerosol forcing can fit observed global warming. The GISS (2014) model (ECS = 2.6°C) with IPCC AR6 aerosol forcing can match observed warming ( Fig. 19 ) in the last half century (when human-made climate forcing overwhelmed natural forcings, unforced climate variability, and flaws in observations). However, agreement also can be achieved by climate models with high ECS. The GISS (2020) model (with ECS = 3.5°C) yields greater warming than observed if IPCC aerosol forcing is used, but less than observed for the alternative aerosol scenario ( Fig. 19 ). This latter aerosol scenario achieves agreement with observed warming if ECS ∼4°C (green curve in Fig. 19 ). 12 Agreement can be achieved with even higher ECS by use of a still more negative aerosol forcing.
![abstract for global warming research paper Global temperature change TG due to aerosols + GHGs calculated with Green’s function Equation (5) using GISS (2014) and GISS (2020) response functions (Fig. 4). Observed temperature is the NASA GISS analysis [155, 156]. Base period: 1951–1980 for observations and model.](https://oup.silverchair-cdn.com/oup/backfile/Content_public/Journal/oocc/3/1/10.1093_oxfclm_kgad008/1/m_kgad008f19.jpeg?Expires=1726813425&Signature=KgcF0d2imu5WPKh8ptnNEW0LWpjuKB~v0nhhnRO6JI2GXXsHD1twtYLnLjVrFWXiVUm9If5hJMa0PLZfhmOuPGjZKBFMGjRY8POnNJXT0YDnwQyhTw3-7wZJzlpCXu3Kiu5FXjzjORGQNKXEuku6ZIOA9V9y8Yd-6pWgCkVRb5tTuoCUuduJEDit02KLCYJacqnZP0VvnqKCYHSg~vREZ0CQzS7nwfB8HAKihKvpJXgIdm7zwUnsYPDRDHsfUKxlwYN4aLy9CCN1aVzrwuyHNBD0QYT6hAT3a2LkXzwBIswIyTrFEhMfjFUCr6Y78AL1xP87uurEa39bmey5tkNH-A__&Key-Pair-Id=APKAIE5G5CRDK6RD3PGA)
Global temperature change T G due to aerosols + GHGs calculated with Green’s function Equation (5) using GISS (2014) and GISS (2020) response functions ( Fig. 4 ). Observed temperature is the NASA GISS analysis [ 155 , 156 ]. Base period: 1951–1980 for observations and model.
The issue we raise is the magnitude of the aerosol forcing, with implications for future warming when particulate air pollution is likely to be reduced. We suggest that IPCC reports may have gravitated toward climate sensitivity near 3°C for 2 × CO 2 in part because of difficulty that models have in realistically simulating amplifying cloud feedbacks and a climate model tendency for excessive mixing of heat into the deep ocean. Our finding from paleoclimate analysis that ECS is 1.2°C ± 0.3°C per W/m 2 (4.8°C ± 1.2°C for 2 × CO 2 ) implies that the (unmeasured) aerosol forcing must be more negative than IPCC’s best estimate. In turn—because aerosol-cloud interactions are the main source of uncertainty in aerosol forcing—this finding emphasizes the need to measure both global aerosol and cloud particle properties.
The case for monitoring global aerosol climate forcing will grow as recognition of the need to slow and reverse climate change emerges. Aerosol and cloud particle microphysics must be measured with precision adequate to define the forcing [ 142 , 158 ]. In the absence of such Keeling-like global monitoring, progress can be made via more limited satellite measurements of aerosol and cloud properties, field studies, and aerosol and cloud modeling. As described next, a great opportunity to study aerosol and cloud physics is provided by a recent change in the IMO (International Maritime Organization) regulations on ship emissions.
The great inadvertent aerosol experiment
Sulfate aerosols are cloud condensation nuclei (CCN), so sulfate emissions by ships result in a larger number of smaller cloud particles, thus affecting cloud albedo and cloud lifetime [ 144 ]. Ships provide a large percentage of sulfates in the North Pacific and North Atlantic regions ( Fig. 20 ). It has been suggested that cooling by these clouds is overestimated because of cloud liquid water adjustments [ 159 ], but Manshausen et al . [ 160 ] present evidence that liquid water path (LWP) effects are substantial even in regions without visible ship-tracks; they estimate a LWP forcing −0.76 ± 0.27 W/m 2 , in stark contrast with the IPCC estimate of +0.2 ± 0.2 W/m 2 . Wall et al . [ 161 ] use satellite observations to quantify relationships between sulfates and low-level clouds; they estimate a sulfate indirect aerosol forcing of −1.11 ± 0.43 W/m 2 over the global ocean. The range of aerosol forcings used in CMIP6 and AR6 GCMs (small blue bar in Fig. 18 ) is not a measure of aerosol forcing uncertainty. The larger bar, from Chapter 7 [ 162 ] of AR6, has negative forcing as great as –2 W/m 2 , but even that does not measure the full uncertainty.
![abstract for global warming research paper Total sulfate (parts per trillion by volume) and percentage of total sulfate provided by shipping in simulations of Jin et al. [157] prior to IMO regulations on sulfur content of fuels.](https://oup.silverchair-cdn.com/oup/backfile/Content_public/Journal/oocc/3/1/10.1093_oxfclm_kgad008/1/m_kgad008f20.jpeg?Expires=1726813425&Signature=Bus1ue0WIV1JPafEilkbXNCz7r7fhY0OFh5jjOctyVTO~K7esGL2eMsxewCnPMTzRMoPUQml5fyH3ybcZhEq1Xar1axNygIsSTIZt8GSfA2kJep4BEiFk2uYHcN170vRI~qhdVC~2wPF9feQHpqOIr48aeBDA7MGjjuOiDVAbGlvcI4M-4dXGkvm5BPLwGSfZeO5J7mki1b~C6u59dJG~QVw5yahgLiU3SlZSwS4NUc9jTxbI-i-hm6ZXN6jAsCGqLP8WS2PDOKwZb1JhGFgF39srmLbwmE~IgSCyls5YB~vVZI5btWeGiQDXIz4T5PEI8pJ9hWcyh-MrC2oVg7c2g__&Key-Pair-Id=APKAIE5G5CRDK6RD3PGA)
Total sulfate (parts per trillion by volume) and percentage of total sulfate provided by shipping in simulations of Jin et al . [ 157 ] prior to IMO regulations on sulfur content of fuels.
Changes of IMO emission regulations provide a great opportunity for insight into aerosol climate forcing. Sulfur content of fuels was limited to 1% in 2010 near the coasts of North America and in the North Sea, Baltic Sea and English Channel, and further restricted there to 0.1% in 2015 [ 163 ]. In 2020 a limit of 0.5% was imposed worldwide. The 1% limit did not have a noticeable effect on ship-tracks, but a striking reduction of ship-tracks was found after the 2015 IMO regulations, especially in the regions near land where emissions were specifically limited [ 164 ]. Following the additional 2020 regulations [ 165 ], global ship-tracks were reduced more than 50% [ 166 ].
Earth’s albedo (reflectivity) measured by CERES (Clouds and Earth’s Radiant Energy System) satellite-borne instruments [ 81 ] over the 22-years March 2000 to March 2022 reveal a decrease of albedo and thus an increase of absorbed solar energy coinciding with the 2015 change of IMO emission regulations. Global absorbed solar energy is + 1.05 W/m 2 in the period January 2015 through December 2022 relative to the mean for the first 10 years of data ( Fig. 21 ). This increase is 5 times greater than the standard deviation (0.21 W/m 2 ) of annual absorbed solar energy in the first 10 years of data and 4.5 times greater than the standard deviation (0.23 W/m 2 ) of CERES data through December 2014. The increase of absorbed solar energy is notably larger than estimated potential CERES instrument drift, which is <0.085 W/m 2 per decade [ 81 ]. Increased solar energy absorption occurred despite 2015–2020 being the declining phase of the ∼11-year solar irradiance cycle [ 167 ]. Nor can increased absorption be attributed to correlation of Earth’s albedo (and absorbed solar energy) with the Pacific Decadal Oscillation (PDO): the PDO did shift to the positive phase in 2014–2017, but it returned to the negative phase in 2017–2022 [ 168 ].

Global absorbed solar radiation (W/m 2 ) relative to mean of the first 120 months of CERES data. CERES data are available at http://ceres.larc.nasa.gov/order_data.php .
Given the large increase of absorbed solar energy, cloud changes are likely the main cause. Quantitative analysis [ 168 ] of contributions to the 20-year trend of absorbed solar energy show that clouds provide most of the change. Surface albedo decrease due to sea ice decline contributes to the 20-year trend in the Northern Hemisphere, but that sea ice decline occurred especially in 2007, with minimum sea ice cover reached in 2012; over the past decade as global and hemispheric albedos declined, sea ice had little trend [ 169 ]. Potential causes of the cloud changes include: (1) reduced aerosol forcing, (2) cloud feedbacks to global warming, (3) natural variability [ 170 ]. Absorbed solar energy was 0.77 W/m 2 greater in Jan2015-Dec2022 than in the first decade of CERES data at latitudes 20–60°S ( Fig. 22 ), a region of relatively little ship traffic. This change is an order of magnitude larger than the estimate of potential detector degradation [ 81 ].

Absorbed solar radiation for indicated regions relative to first 120 months of CERES data. Southern Hemisphere 20–60°S is 89% ocean. North Atlantic is (20–60°N, 0–60°W) and North Pacific is (20–60°N, 120–220°W). Data source: http://ceres.larc.nasa.gov/order_data.php .
Climate models predict a reduction of cloud albedo in this region as a feedback effect driven by global warming [ 12 ] (Sec. 7.4.2.4). Continued monitoring of absorbed energy can confirm the reality of the change, but without global monitoring of detailed physical properties of aerosols and clouds [ 142 ], it will be difficult to apportion observed change among candidate causes.
North Pacific and North Atlantic regions of heavy ship traffic are ripe for detailed study of cloud changes and their causes, although unforced cloud variability is large in such sub-global regions. Both regions have increased absorption of solar radiation after 2015 ( Fig. 22 ). The 2014–2017 maximum absorption in the North Pacific is likely enhanced by reduced cloud cover during the positive PDO, but the more recent high absorption is during the negative PDO phase. In the North Atlantic, persistence of increased absorption for several years exceeds prior variability, but longer records plus aerosol and cloud microphysical data are needed for interpretation.
Climate change is characterized by delayed response and amplifying feedbacks. Delayed response makes human-made climate forcing a threat to today’s public and future generations because of the practical difficulty of reversing the forcing once consequences become apparent. Feedbacks determine climate sensitivity to any applied forcing. We find that Earth’s climate is very sensitive—more sensitive than the best estimate of the Intergovernmental Panel on Climate Change (IPCC)—which implies that there is a great amount of climate change ‘in the pipeline.’ Extraordinary actions are needed to reduce the net human-made climate forcing, as is required to reduce global warming and avoid highly undesirable consequences for humanity and nature.
Equilibrium climate sensitivity (ECS)
The 1979 Charney study [ 4 ] considered an idealized climate sensitivity in which ice sheets and non-CO 2 GHGs are fixed. The Charney group estimated that the equilibrium response to 2 × CO 2 , a forcing of 4 W/m 2 , was 3°C, thus an ECS of 0.75°C per W/m 2 , with one standard deviation uncertainty σ = 0.375°C. Charney’s estimate stood as the canonical ECS for more than 40 years. The current IPCC report [ 12 ] concludes that 3°C for 2 × CO 2 is their best estimate for ECS.
We compare recent glacial and interglacial climates to infer ECS with a precision not possible with climate models alone. Uncertainty about Last Glacial Maximum (LGM) temperature has been resolved independently with consistent results by Tierney et al . [ 49 ] and Seltzer et al . [ 51 ]. The Tierney approach, using a collection of geochemical temperature indicators in a global analysis constrained by climate change patterns defined by a global climate model, is used by Osman et al . [ 50 ] to find peak LGM cooling 7.0 ± 1°C (2σ, 95% confidence) at 21–18 kyBP. We show that, accounting for polar amplification, these analyses are consistent with the 5.8 ± 0.6°C LGM cooling of land areas between 45°S and 35°N found by Seltzer et al . using the temperature-dependent solubility of dissolved noble gases in ancient groundwater. The forcing that maintained the 7°C LGM cooling was the sum of 2.25 ± 0.45 W/m 2 (2σ) from GHGs and 3.5 ± 1.0 W/m 2 (2σ) from the LGM surface albedo, thus 5.75 ± 1.1 W/m 2 (2σ). ECS implied by the LGM is thus 1.22 ± 0.29°C (2σ) per W/m 2 , which, at this final step, we round to 1.2 ± 0.3°C per W/m 2 . For transparency, we have combined uncertainties via simple RMS (root-mean-square). ECS as low as 3°C for 2 × CO 2 is excluded at the 3σ level, i.e. with 99.7% confidence.
More sophisticated mathematical analysis, which has merits but introduces opportunity for prior bias and obfuscation, is not essential; error assessment ultimately involves expert judgment. Instead, focus is needed on the largest source of error: LGM surface albedo change, which is uncertain because of the effect of cloud shielding on the efficacy of the forcing. As cloud modeling is advancing rapidly, this topic is ripe for collaboration of CMIP [ 53 ] (Coupled Model Intercomparison Project) with PMIP [ 54 ] (Paleoclimate Modelling Intercomparison Project). Simulations should include at the same time change of surface albedo and topography of ice sheets, vegetation change, and exposure of continental shelves due to lower sea level.
Knowledge of climate sensitivity can be advanced further via analysis of the wide climate range in the Cenozoic era (Earth system sensitivity section). However, interpretation of data and models, and especially projections of climate change, depend on understanding of climate response time.
We expected climate response time—the time for climate to approach a new equilibrium after imposition of a forcing—to become faster as mixing of heat in ocean models improved [ 75 ]. That expectation was not met when we compared two generations of the GISS GCM (global climate model). The GISS (2020) GCM is improved [ 32 , 33 ] in its ocean simulation over the GISS (2014) GCM as a result of higher vertical and horizontal resolution, more realistic parameterization of sub-grid scale motions, and correction of errors in the ocean computer program [ 32 ]. Yet the time for the model to achieve 63% of its equilibrium response remained about 100 years. There are two reasons for this: one that is obvious and one that is more interesting and informative.
The surface in the newer model warms as fast as in the older model, but it must achieve greater warming to reach 63% of equilibrium because its ECS is higher, which is one reason that the response time remains long. The other reason is that Earth’s energy imbalance (EEI) in the newer model decreases rapidly. EEI defines the rate that heat is pumped into the ocean, so a smaller EEI implies a longer time for the ocean to reach its new equilibrium temperature. Quick drop of EEI—in the first year after introduction of the forcing—implies existence of ultrafast feedback in the GISS (2020) model. For want of an alternative with such a large effect on Earth’s energy budget, we infer a rapid cloud feedback and we suggest (Slow, fast and ultrafast feedbacks section) a set of brief GCM runs that define cloud changes and other diagnostic quantities to an arbitrary accuracy.
The Charney report [ 4 ] recognized that clouds were a main cause of a wide range in ECS estimates. Today, clouds still cast uncertainty on climate predictions. Several CMIP6 [ 34 ] GCMs have ECS of ∼4–6°C for 2×CO 2 [ 171 , 172 ] with the high sensitivity caused by cloud feedbacks [ 84 ]. As cloud modeling progresses, it will aid understanding if climate models report their 2 × CO 2 response functions for both temperature and EEI (Earth’s energy imbalance).
Fast EEI response—faster than global temperature response—has a practical effect: observed EEI understates the reduction of climate forcing required to stabilize climate. Although the magnitude of this effect is uncertain (see Supplementary Material SM6 ), it makes the task of restoring a hospitable climate and saving coastal cities more challenging. On the other hand, long climate response time implies the potential for educated policies to affect the climate outcome before the most undesirable consequences occur.
The time required for climate to reach a new equilibrium is relevant to policy (Perspective on policy implications section), but there is another response time of practical importance. With climate in a state of disequilibrium, how much time do we have before we pass the point of no return, the point where major climate impacts are locked in, beyond our ability to control? That’s a complex matter; it requires understanding of ‘slow’ feedbacks, especially ice sheets. It also depends on how far climate is out of equilibrium. Thus, we first consider the full Earth system sensitivity.
Earth system sensitivity (ESS)
The Cenozoic era—the past 66 million years—provides an opportunity to study Earth system sensitivity via a consistent analysis for climate ranging from hothouse conditions with Earth 15°C warmer and sea level 60 m higher than preindustrial climate to glacial conditions with Earth 7°C cooler and sea level 120 m lower than preindustrial. Atmospheric CO 2 amount in the past 800 000 years ( Fig. 2 ), confirms expectation that CO 2 is the main control knob [ 87 ] on global temperature. We can assume this control existed when CO 2 amount varied due to CO 2 emissions caused by plate tectonics (continental drift). The two-step [ 91 ] that the Indian plate executed as it moved through the Tethys (now Indian) ocean left a signature in atmospheric CO 2 and global temperature. CO 2 emissions from subduction of ocean crust were greatest when the Indian plate was moving fastest (inset, Fig. 6 ) and peaked at its hard collision with the Eurasian plate at 50 MyBP. Diminishing metamorphic CO 2 emissions continue as the Indian plate is subducted beneath the Eurasian plate, pushing up the Himalayan Mountains, but carbon drawdown from weathering and burial of organic carbon exceeds emissions. Motion of the Indian Plate thus dominates the broad sweep of Cenozoic CO 2 , but igneous provinces play a role. The North Atlantic Igneous Province (caused by a rift in the sea floor as Greenland pulled away from Europe) that triggered the Paleocene-Eocene Thermal Maximum (PETM) event about 56 MyBP and the Columbia River Flood Basalt about 15 MyBP ( Fig. 6 ) are most notable.
We infer the Cenozoic history of sea surface temperature (SST) at sites of deepwater formation from the oxygen isotope δ 18 O in shells of deep-ocean-dwelling foraminifera preserved in ocean sediments [ 44 , 90 ]. High latitude SST change—including a correction term as SST approaches the freezing point—provides an accurate estimate of global surface temperature change. This Cenozoic temperature history and climate sensitivity inferred from the LGM cooling yield an estimate of Cenozoic CO 2 history. We suggest that this whole-Cenozoic approach may define the CO 2 history ( Fig. 9a ) more accurately than CO 2 proxy measurements. We find CO 2 about 325 ppm in the early Pliocene and 450 ppm at transition to glaciated Antarctica. Global climate models (GCMs) that isolate on the Pliocene tend to use CO 2 levels of order 400 ppm in attempts to match actual Pliocene warmth and ice sheet models use CO 2 of order 700 ppm or greater to achieve ice sheet disintegration on Antarctica, which suggests that the models are not realistically capturing amplifying feedback processes (see Cenozoic CO 2 section).
The Cenozoic provides a perspective on present greenhouse gas (GHG) levels. The dashed line in Fig. 23 is the ‘we are here’ level of GHG climate forcing. Today’s GHG forcing of 4.6 W/m 2 is relative to mid-Holocene CO 2 of 260 ppm; we present evidence in Cenozoic CO 2 section that 260 ppm is the natural Holocene CO 2 level. Human-caused GHG forcing today is already above the level needed to deglaciate Antarctica, if such forcing is left in place long enough. We do not predict full deglaciation of Antarctica on a time scale people care about—rather we draw attention to how far today’s climate is out of equilibrium with today’s GHG level. This is one measure of how strongly humanity is pushing the climate system. Stabilizing climate requires removing the disequilibrium by reducing human-made climate forcing. A danger is that it will become difficult or implausible to prevent large sea level rise, if deglaciation is allowed to get well underway.

Forcing required to yield Cenozoic temperature for today’s solar irradiance, compared with human-made GHG forcing in 2022.
GHGs are not the only large human-made climate forcing. Understanding of ongoing climate change requires that we also include the effect of aerosols (fine airborne particles).
Aerosol climate forcing is larger than the IPCC AR6 estimate and has likely been significant for millennia. We know of no other persuasive explanation for absence of global warming in the last half of the Holocene ( Fig. 14 ) as GHG forcing increased 0.5 W/m 2 ( Fig. 15 ). Climate models without a growing negative aerosol forcing yield notable warming in that period [ 173 ], a warming that, in fact, did not occur. Negative aerosol forcing, increasing as civilization developed and population grew, is expected. As humans burned fuels at a growing rate—wood and other biomass for millennia and fossil fuels in the industrial era—aerosols as well as GHGs were an abundant, growing, biproduct. The aerosol source from wood-burning has continued in modern times [ 146 ]. GHGs are long-lived and accumulate, so their forcing dominates eventually, unless aerosol emissions grow higher and higher—the Faustian bargain [ 98 ].
Multiple lines of evidence show that aerosol forcing peaked early this century [ 174 ]. Emissions from the largest sources, China and India, were increasing in 2000, but by 2010 when the first limits on ship emissions were imposed, China’s emissions were declining. We estimate peak (negative) aerosol forcing as at least 1.5–2 W/m 2 , with turning point at 2010, consistent with Fig. 3 of Bauer et al . [ 175 ] GHG plus aerosol forcing grew +0.3 W/m 2 per decade (GHGs: +0.45, aerosols: –0.15) during 1970–2010, which produced warming of 0.18°C per decade. With current policies, we expect climate forcing for a few decades post-2010 to increase 0.5–06 W/m 2 per decade and produce global warming of at least +0.27°C per decade. In that case, global warming will reach 1.5°C in the 2020s and 2°C before 2050 ( Fig. 24 ). Such acceleration is dangerous in a climate system that is already far out of equilibrium and dominated by multiple amplifying feedbacks.

Global temperature relative to 1880–1920. Edges of the predicted post-2010 accelerated warming rate (see text) are 0.36 and 0.27°C per decade.
The sharp change of ship emissions in 2020 (The great inadvertent aerosol experiment section) provides an indirect measure of aerosol effects. Diamond [ 176 ] finds a cloud brightness decrease of order 1 W/m 2 in a shipping corridor. We find a larger effect, increased absorption of about 3 W/m 2 in regions of heavy ship traffic in the North Atlantic and North Pacific ( Fig. 22 ), but a longer record is needed to define significance. However, the single best sentinel for global climate change is Earth’s energy imbalance.
Earth’s energy imbalance
Earth’s energy imbalance (EEI) is the net gain (or loss) of energy by the planet, the difference between absorbed solar energy and emitted thermal (heat) radiation. As long as EEI is positive, Earth will continue to get hotter. EEI is hard to measure, a small difference between two large quantities (Earth absorbs and emits about 240 W/m 2 averaged over the entire planetary surface), but change of EEI can be well-measured from space [ 81 ]. Absolute calibration is from the change of heat in the heat reservoirs, mainly the global ocean, over a period of at least a decade, as needed to reduce error due to the finite number of places that the ocean is sampled [ 80 ]. EEI varies year-to-year ( Fig. 25 ), largely because global cloud amount varies with weather and ocean dynamics, but averaged over several years EEI helps inform us about what is needed to stabilize climate.
![abstract for global warming research paper 12-month running-mean of Earth’s energy imbalance from CERES satellite data [81] normalized to 0.71 W/m2 mean for July 2005–June 2015 (blue bar) from in situ data [80].](https://oup.silverchair-cdn.com/oup/backfile/Content_public/Journal/oocc/3/1/10.1093_oxfclm_kgad008/1/m_kgad008f25.jpeg?Expires=1726813425&Signature=oJcvBXREylBgyrFrkV9WuqKYko3YchukBXoomqDc1sdG0sj~eCvI3R6~Z9rLtAHDhEudCL54lvNXXzedS8jO3p1Xx5X-Zewkg2~FOPRESbHwOkQdogWYq5z286623gxD06uG0rxDpngi2EG4qic0ba3oKJdmvYeVI2UrrcfGW6wQKDUcdQa3db3jZHGZLzrPPKJLcZiAXeroQg4CFyjDNzpCh3GYeC4xJ2eBomRx-qttd7JyVoTFOoQfxzmfh6HLfKtxFceIA7VNrL1SULsn0IEJimxAPnDxMhwq2~AmnuLOCayg2ljzFWHtOrXVUP-pgNsU2c9VxGwp0CtcgFHSUg__&Key-Pair-Id=APKAIE5G5CRDK6RD3PGA)
12-month running-mean of Earth’s energy imbalance from CERES satellite data [ 81 ] normalized to 0.71 W/m 2 mean for July 2005–June 2015 (blue bar) from in situ data [ 80 ].
The data indicate that EEI has doubled since the first decade of this century ( Fig. 25 ). This increase is one basis for our prediction of post-2010 acceleration of the global warming rate. The EEI increase may be partly due to restrictions on maritime aerosol precursor emissions imposed in 2015 and 2020 (The great inadvertent aerosol experiment section), but the growth rate of GHG climate forcing also increased in 2015 and since has remained at the higher level (Equilibrium warming versus committed warming section).
Reduction of climate forcing needed to reduce EEI to zero is greater than EEI because of ultrafast cloud feedback (Slow, fast and ultrafast feedbacks section), but the magnitude of this effect is uncertain (SM6). Cloud feedbacks are only beginning to be simulated well, but climate sensitivity near 1.2°C per W/m 2 implies that the net cloud feedback is large and deserves greater attention. Precise monitoring of EEI is essential as a sentinel for future climate change and to assess efforts to stabilize climate and avoid undesirable consequences. Global satellite monitoring of geographical and temporal changes of EEI and ocean in situ monitoring (especially in polar regions of rapid change) are both needed for the sake of understanding ongoing climate change.
Equilibrium warming versus committed warming
Equilibrium warming for today’s climate forcing is the warming required to restore Earth’s energy balance if atmospheric composition is fixed at today’s conditions. Equilibrium warming is a benchmark that can be evaluated from atmospheric composition and paleoclimate data, with little involvement of climate models. It is the standard benchmark used in definition of the Charney ECS (equilibrium climate sensitivity excluding slow feedbacks) [ 4 ] and ESS (Earth system sensitivity, which includes slow feedbacks such as ice sheet size) [ 71 ]. GHG climate forcing now is 4.6 W/m 2 relative to the mid-Holocene (7 kyBP) or 4.1 W/m 2 relative to 1750. There is little merit in debating whether GHG forcing is 4.6 or 4.1 W/m 2 because it is still increasing 0.5 W/m 2 per decade (Perspective on policy implications section). ECS response to 4.6 W/m 2 forcing for climate sensitivity 1.2°C per W/m 2 is 5.5°C. The eventual Earth system response (ESS) to sustained 4.6 W/m 2 forcing is about 10°C (Earth system sensitivity section), because that forcing is large enough to deglaciate Antarctica ( Fig. 23 ). Net human-made forcing today is probably near 3 W/m 2 due to negative aerosol forcing. Even 3 W/m 2 may be sufficient to largely deglaciate Antarctica, if the forcing is left in place permanently ( Fig. 23 ).
‘Committed warming’ is less precisely defined; even in the current IPCC report [ 12 ] (p. 2222) it has multiple definitions. One concept is the warming that occurs if human-made GHG emissions cease today, but that definition is ill-posed as well as unrealistic. Do aerosol emissions also cease? That would cause a sudden leap in Earth’s energy imbalance, a ‘termination shock,’ as the cooling effect of human-made aerosols disappears. A more useful definition is the warming that will occur with plausibly rapid phasedown of GHG emissions, including comparison with ongoing reality. However, the required ‘integrated assessment models,’ while useful, are complex and contain questionable assumptions that can mislead policy (see Perspective on policy implications section).
Nature’s capacity for restoration provides hope that future warming can be limited, if humanity moves promptly toward sustainable energy and climate policies. Earth’s ability to remove human-made CO 2 emissions from the atmosphere is revealed by Fig. 26 . Fossil fuel emissions now total more than 10 GtC/year, which is almost 5 ppm of CO 2 , yet CO 2 in the air is only increasing 2.5 ppm/year. The other half is being taken up by the ocean, solid land, and biosphere. Indeed, Earth is taking up even more because deforestation, fires, and poor agricultural and forestry practices are additional human-made CO 2 sources. If human emissions ceased, atmospheric CO 2 would initially decline a few ppm per year, but uptake would soon slow—it would take millennia for CO 2 to reach preindustrial levels [ 131 ]. This underscores the difficulty of restoring Earth’s energy balance via emission reductions alone. Furthermore, fossil fuels have raised living standards in most of the world and still provide 80% of the world’s energy, which contributes to a policy inertia. As the reality of climate change emerges, the delayed response of climate and amplifying feedbacks assure that the world has already set sail onto even more turbulent climate seas. Scientists must do their best to help the public understand policy options that may preserve and restore a propitious climate for future generations.

Fossil fuel emissions divided into portions appearing in the annual increase of airborne CO 2 and the remainder, which is taken up by the ocean and land (1 ppm CO 2 ∼ 2.12 GtC).
This section is the first author’s perspective based on more than 20 years of experience on policy issues that began with a paper [ 179 ] and two workshops [ 180 ] that he organized at the East-West Center in Hawaii, followed by meetings and workshops with utility experts and trips to more than a dozen nations for discussions with government officials, energy experts, and environmentalists. The aim was to find a realistic scenario with a bright energy and climate future, with emphasis on cooperation between the West and nations with emerging or underdeveloped economies.
Energy, CO 2 and the climate threat
The world’s energy and climate path has good reason: fossil fuels powered the industrial revolution and raised living standards. Fossil fuels still provide most of the world’s energy ( Fig. 27a ) and produce most CO 2 emissions ( Fig. 27b ). Much of the world is still in early or middle stages of economic development. Energy is needed and fossil fuels are a convenient, affordable source of energy. One gallon (3.8 l) of gasoline (petrol) provides the work equivalent of more than 400 h labor by a healthy adult. These benefits are the basic reason for continued high emissions. The Covid pandemic dented emissions in 2020, but 2022 global emissions were a record high level. Fossil fuel emissions from mature economies are beginning to fall due to increasing energy efficiency, introduction of carbon-free energies, and export of manufacturing from mature economies to emerging economies. However, at least so far, those reductions have been more than offset by increasing emissions in developing nations ( Fig. 28 ).
![abstract for global warming research paper Global energy consumption and CO2 emissions (Hefner at al. [177] and Energy Institute [178]).](https://oup.silverchair-cdn.com/oup/backfile/Content_public/Journal/oocc/3/1/10.1093_oxfclm_kgad008/1/m_kgad008f27.jpeg?Expires=1726813425&Signature=kjClLB9E-m4JzT~StFdr6fQM89lR4uyUQKMLulA-iUU7DF3lP-dZgN0rRHnUQTrKtOjoka5l3cNh7wl~syP4XxKe-UC9FxVznEpJ8oKXyuo0AajcL7a48PeDcvwW0iBTHm9Qlba6tKLtrC1pu~A1K5nMQRdQuiz6fCZJgbQES7Az3WRMFOAlRJ5i3tx6VfqIcJhrCgslwkXFIRfWeiQS-WdDZUuyw9Oz87jvXsMDMJFaT0FCkaFvfcfHffCU~Qv3hkhTjto8ftVu0QVuiRahtENFjq2qg-G172ZIn47cAadtwzF6Ao6ybsEsPFn-lqA77bUqlNpfL6aa0oKFA-s4gg__&Key-Pair-Id=APKAIE5G5CRDK6RD3PGA)
Global energy consumption and CO 2 emissions (Hefner at al . [ 177 ] and Energy Institute [ 178 ]).

Fossil fuel CO 2 emissions from mature and emerging economies. China is counted as an emerging economy. Data sources as in Fig. 27 .
The potential for rising CO 2 to be a serious threat to humanity was the reason for the 1979 Charney report, which confirmed that climate was likely sensitive to expected CO 2 levels in the 21st century. In the 1980s it emerged that high climate sensitivity implied a long delay between changing atmospheric composition and the full climate response. Ice core data revealed the importance of amplifying climate feedbacks. A climate characterized by delayed response and amplifying feedbacks is especially dangerous because the public and policymakers are unlikely to make fundamental changes in world energy systems until they see visible evidence of the threat. Thus, it is incumbent on scientists to make this situation clear to the public as soon as possible. That task is complicated by the phenomenon of scientific reticence.
Scientific reticence
Bernard Barber decried the absence of attention to scientific reticence, a tendency of scientists to resist scientific discovery or new ideas [ 139 ]. Richard Feynman needled fellow physicists about their reticence to challenge authority [ 181 ], specifically to correct the electron charge that Millikan derived in his famous oil drop experiment. Later researchers moved Millikan’s result bit by bit—experimental uncertainties allow judgment—reaching an accurate result only after years. Their reticence embarrassed the physics community but caused no harm to society. A factor that may contribute to reticence among climate scientists is ‘delay discounting:’ preference for immediate over delayed rewards [ 182 ]. The penalty for ‘crying wolf’ is immediate, while the danger of being blamed for ‘fiddling while Rome was burning’ is distant. One of us has noted [ 183 ] that larding of papers and proposals with caveats and uncertainties increases chances of obtaining research support. ‘Gradualism’ that results from reticence is comfortable and well-suited for maintaining long-term support. Gradualism is apparent in IPCC’s history in evaluating climate sensitivity as summarized in our present paper. Barber identifies professional specialization—which causes ‘outsiders’ to be ignored by ‘insiders’—as one cause of reticence; specialization is relevant to ocean and ice sheet dynamics, matters upon which the future of young people hangs.
Discussion [ 184 ] with field glaciologists 13 20 years ago revealed frustration with IPCC’s ice sheet assessment. One glaciologist said—about a photo [ 185 ] of a moulin (a vertical shaft that carries meltwater to the base of the Greenland ice sheet)—‘the whole ice sheet is going down that damned hole!’ Concern was based on observed ice sheet changes and paleoclimate evidence of sea level rise by several meters in a century, implying that ice sheet collapse is an exponential process. Thus, as an alternative to ice sheet models, we carried out a study described in Ice Melt [ 13 ]. In a GCM simulation, we added a growing freshwater flux to the ocean surface mixed layer around Greenland and Antarctica, with the flux in the early 21st century based on estimates from in situ glaciological studies [ 186 ] and satellite data on sea level trends near Antarctica [ 187 ]. Doubling times of 10 and 20 years were used for the growth of freshwater flux. One merit of our GCM was reduced, more realistic, small-scale ocean mixing, with a result that Antarctic Bottom Water formed close to the Antarctic coast [ 13 ], as in the real world. Growth of meltwater and GHG emissions led to shutdown of the North Atlantic and Southern Ocean overturning circulations, amplified warming at the foot of the ice shelves that buttress the ice sheets, and other feedbacks consistent with ‘nonlinearly growing sea level rise, reaching several meters in 50–150 years’ [ 13 ]. Shutdown of ocean overturning circulation occurs this century, as early as midcentury. The 50–150-year time scale for multimeter sea level rise is consistent with the 10–20-year range for ice melt doubling time. Real-world ice melt will not follow a smooth curve, but its growth rate is likely to accelerate in coming years due to increasing heat flux into the ocean ( Fig. 25 ).
We submitted Ice Melt to a journal that makes reviews publicly available [ 188 ]. One reviewer, an IPCC lead author, seemed intent on blocking publication, while the other reviewer described the paper as a ‘masterwork of scholarly synthesis, modeling virtuosity, and insight, with profound implications’. Thus, the editor obtained additional reviewers, who recommended publication. Promptly, an indictment was published [ 189 ] of our conclusion that continued high GHG emissions would cause shutdown of the AMOC (Atlantic Meridional Overturning Circulation) this century. The 15 authors, representing leading GCM groups, used 21 climate projections from eight ‘…state-of-the-science, IPCC class…’ GCMs to conclude that ‘…the probability of an AMOC collapse is negligible. This is contrary to a recent modeling study [ Hansen et al . , 2016 ] that used a much larger, and in our assessment unrealistic, Northern Hemisphere freshwater forcing… According to our probabilistic assessment, the likelihood of an AMOC collapse remains very small (<1% probability) if global warming is below ∼ 5K…’[ 189 ]. They treated the ensemble of their model results as if it were the probability distribution for the real world.
In contrast, we used paleoclimate evidence, global modeling, and ongoing climate observations. Paleoclimate data [ 190 ] showed that AMOC shutdown is not unusual and occurred in the Eemian (when global temperature was similar to today), and also that sea level in the Eemian rose a few meters within a century [ 191 ] with the likely source being collapse of the West Antarctic ice sheet. Although we would not assert that our model corrected all excessive ocean mixing, the higher vertical resolution and improved mixing increased the sensitivity to freshwater flux, as confirmed in later tests [ 192 ]. Modern observations showed and continue to add evidence that the overturning Southern Ocean [ 193 , 194 ] and North Atlantic [ 195 ] are already slowing. Growth of meltwater injection onto the Southern [ 196 ] and North Atlantic Oceans [ 197 ] is consistent with a doubling time of 10–20 years. High climate sensitivity inferred in our present paper also implies there will be a greater increase of precipitation on polar oceans than that in most climate models.
The indictment of Ice Melt by Bakker et al . [ 189 ] was accepted by the research community. Papers on the same topics ignored our paper or referred to it parenthetically with a note that we used unrealistic melt rates, even though these were based on observations. Ice Melt was blackballed in IPCC’s AR6 report, which is a form of censorship [ 14 ]. Science usually acknowledges alternative views and grants ultimate authority to nature. In the opinion of our first author, IPCC does not want its authority challenged and is comfortable with gradualism. Caution has merits, but the delayed response and amplifying feedbacks of climate make excessive reticence a danger. Our present paper—via revelation that the equilibrium response to current atmospheric composition is a nearly ice-free Antarctica—amplifies concern about locking in nonlinearly growing sea level rise. Also, our conclusion that CO 2 was about 450 ppm at Antarctic glaciation disparages ice sheet models. Portions of the ice sheets may be recalcitrant to rapid change, but enough ice is in contact with the ocean to provide of the order of 25 m (80 feet) of sea level rise. Thus, if we allow a few meters of sea level rise, we may lock in much larger sea level rise.
Climate change responsibilities
The industrial revolution began in the U.K., which was the largest source of fossil fuel emissions in the 19th century ( Fig. 29a ), but development soon moved to Germany, the rest of Europe, and the U.S. Nearly half of global emissions were from the U.S. in the early 20th century, and the U.S. is presently the largest source of cumulative emissions ( Fig. 29b ) that drive climate change [ 198 , 199 ]. Mature economies, mainly in the West, are responsible for most cumulative emissions, especially on a per capita basis ( Fig. 30 ). Growth of emissions is now occurring in emerging economies ( Figs 28 and 29a ). China’s cumulative emissions will eventually pass those of the U.S. in the absence of a successful effort to replace coal with carbon-free energy.

Fossil fuel CO 2 emissions by nation or region as a fraction of global emissions. Data sources as in Fig. 27 .
![abstract for global warming research paper Cumulative per capita national fossil fuel emissions [200].](https://oup.silverchair-cdn.com/oup/backfile/Content_public/Journal/oocc/3/1/10.1093_oxfclm_kgad008/1/m_kgad008f30.jpeg?Expires=1726813425&Signature=r~UAJV-NC1RAcMLJuC9HetfRnVQidYnmYW3oKmd8SKpZ4JXBUOKoh6Kssh7NlM28-j9r9x3bdPo5WctEXtSOesGauz77Nkcmn1DVn9F~gzJSAlyuMAHaW2aBSrKUuuL7sN9oP2K0q4-eU3w690A37VW~RfvkfE0z-asC2vRrHYSyR~udlnVyH0gQUsYtCEAe~40~PDR3TtqT-xM1zvBI9IQXexxnIrSsAkhiINRR9nRbv-btJr3RhrRdHjUsQ~0naWDDDIpQEPBJx3~bvVE9MuGu5m~EyWHyd2Uf19bidjYaopZD2nVelPH0Rqv4uLbJCodcoxLBw3BjHhTxHuFpSQ__&Key-Pair-Id=APKAIE5G5CRDK6RD3PGA)
Cumulative per capita national fossil fuel emissions [ 200 ].
Greenhouse gas emissions situation
The United Nations uses a target for maximum global warming to cajole progress in limiting climate change. The 2015 Paris Agreement [ 201 ] aimed to hold ‘the increase in the global average temperature to well below 2°C above the pre-industrial levels and pursue efforts to limit the temperature increase to 1.5°C above the pre-industrial levels.’ The IPCC AR5 report added a climate forcing scenario, RCP2.6, with a rapid decrease of GHG climate forcings, as needed to prevent global warming from exceeding 2°C. Since then, a gap between that scenario and reality opened and is growing ( Fig. 31 ). The 0.03 W/m 2 gap in 2022 could be closed by extracting CO 2 from the air. However, required negative emissions (CO 2 extracted from the air and stored permanently) must be larger than the desired atmospheric CO 2 reduction by a factor of about 1.7 [ 63 ]. Thus, the required CO 2 extraction is 2.1 ppm, which is 7.6 GtC. Based on a pilot direct-air carbon capture plant, Keith [ 202 ] estimates an extraction cost of $450–920 per tC, as clarified elsewhere [ 203 ]. Keith’s cost range yields an extraction cost of $3.4–7.0 trillion. That covers excess emissions in 2022 only; it is an annual cost. Given the difficulty the UN faced in raising $0.1 trillion for climate purposes and the growing emissions gap ( Fig. 31 ), this example shows the need to reduce emissions as rapidly as practical and shows that carbon capture cannot be viewed as the solution, although it may play a role in a portfolio of policies, if its cost is driven down.
![abstract for global warming research paper Annual growth of climate forcing by GHGs [38] including part of O3 forcing not included in CH4 forcing (Supplementary Material). MPTG and OTG are Montreal Protocol and Other Trace Gases.](https://oup.silverchair-cdn.com/oup/backfile/Content_public/Journal/oocc/3/1/10.1093_oxfclm_kgad008/1/m_kgad008f31.jpeg?Expires=1726813425&Signature=f6f2oKLglX7yxXP287tN66Lq8jBIE0Uc0l50q1nPdUj3iwX90RFknBv5Gs1f--Y3G5ygPvBlVS5HdTeRCA~rrmnBaeMQzLBG7YtJI2t5X6Z2qnrCThYByRkNbainxEf45fCWuFp1--OpmQvdgva9fa9Xd9HnliNB0wGbg48-ZK5iOLdTL5GhpKP5MlU3jHnDTm89FFizNCaYoBDPKPc~uXZnCatBEuQTpuacFzjiihOd~CQ5C2MenvWdqs-YWi5O9mWHUZCv44-KNajaPOtp36hICdLQsKUoqfd8O2k1aZIctRaRjWELEz3MAkXbgksrsfY7wS9oANbzECb-INESAg__&Key-Pair-Id=APKAIE5G5CRDK6RD3PGA)
Annual growth of climate forcing by GHGs [ 38 ] including part of O 3 forcing not included in CH 4 forcing ( Supplementary Material ). MPTG and OTG are Montreal Protocol and Other Trace Gases.
IPCC (Intergovernmental Panel on Climate Change), the scientific body advising the world on climate, has not bluntly informed the world that the present precatory policy approach will not keep warming below 1.5°C or even 2°C. The ‘tragedy of the commons’ [ 204 ] is that, as long as fossil fuel pollution can be dumped in the air free of charge, agreements such as the Kyoto Protocol [ 205 ] and Paris Agreement have limited effect on global emissions. Political leaders profess ambitions for dubious net-zero emissions while fossil fuel extraction expands. IPCC scenarios that phase down human-made climate change amount to ‘a miracle will occur’. The IPCC scenario that moves rapidly to negative global emissions (RCP2.6) has vast biomass-burning powerplants that capture and sequester CO 2 , a nature-ravaging, food-security-threatening [ 206 ], proposition without scientific and engineering credibility and without a realistic chance of being deployed at scale and on time to address the climate threat.
Climate and energy policy
Climate science reveals the threat of being too late. ‘Being too late’ refers not only to warning of the climate threat, but also to technical advice on policy implications. Are we scientists not complicit if we allow reticence and comfort to obfuscate our description of the climate situation? Does our training, years of graduate study and decades of experience, not make us well-equipped to advise the public on the climate situation and its policy implications? As professionals with deep understanding of planetary change and as guardians of young people and their future, do we not have an obligation, analogous to the code of ethics of medical professionals, to render to the public our full and unencumbered diagnosis? That is our objective.
The basis for the following opinions of the first author, to the extent not covered in this paper, will be described in a book in preparation [ 2 ]. We are in the early phase of a climate emergency. The present huge planetary energy imbalance assures that climate will become less tolerable to humanity, with greater climate extremes, before it is feasible to reverse the trend. Reversing the trend is essential—we must cool the planet—for the sake of preserving shorelines and saving the world’s coastal cities. Cooling will also address other major problems caused by global warming. We should aim to return to a climate like that in which civilization developed, in which the nature that we know and love thrived. As far as is known, it is still feasible to do that without passing through irreversible disasters such as many-meter sea level rise.
Abundant, affordable, carbon-free energy is essential to achieve a world with propitious climate, while recognizing the rights and aspirations of all people. The staggering magnitude of the task is implied by global and national carbon intensities: carbon emissions per unit energy use ( Fig. 32 ). Global carbon intensity must decline to near zero over the next several decades. This chart—not vaporous promises of net zero future carbon emissions inserted in integrated assessment models—should guide realistic assessment of progress toward clean energy. Policy must include apolitical targeting of support for development of low-cost carbon-free energy. All nations would do well to study strategic decisions of Sweden, which led past decarbonization efforts ( Fig. 32 ) and is likely to lead in the quest for zero or negative carbon intensity that will be needed to achieve a bright future for today’s young people and future generations.

Carbon intensity (carbon emissions per unit energy use) of several nations and the world. Mtoe = megatons of oil equivalent. Data sources as in Fig. 27 .
Given the global situation that we have allowed to develop, three actions are now essential.
First, underlying economic incentives must be installed globally to promote clean energy and discourage CO 2 emissions. Thus, a rising price on GHG emissions is needed, enforced by border duties on products from nations without a carbon fee. Public buy-in and maximum efficacy require the funds to be distributed to the public, which will also address wealth disparity. Economists in the U.S. support carbon fee-and-dividend [ 207 ]; college and high school students join in advocacy [ 208 ]. A rising carbon price creates a level playing field for energy efficiency, renewable energy, nuclear power, and innovations; it would spur the thousands of ‘miracles’ needed for energy transition. However, instead, fossil fuels and renewable energy are now subsidized. Thus, nuclear energy has been disadvantaged and excluded as a ‘clean development mechanism’ under the Kyoto Protocol, based on myths about nuclear energy unsupported by scientific fact [ 209 ]. A rising carbon price is crucial for decarbonization, but not enough. Long-term planning is needed. Sweden provides an example: 50 years ago, its government decided to replace fossil fuel power stations with nuclear energy, which led to its extraordinary and rapid decarbonization ( Fig. 32 ).
Second, global cooperation is needed. De facto cooperation between the West and China drove down the price of renewable energy. Without greater cooperation, developing nations will be the main source of future GHG emissions ( Fig. 28 ). Carbon-free, dispatchable electricity is a crucial need. Nations with emerging economies are eager to have modern nuclear power because of its small environmental footprint. China-U.S. cooperation to develop low-cost nuclear power was proposed, but stymied by U.S. prohibition of technology transfer [ 210 ]. Competition is normal, but it can be managed if there is a will, reaping benefits of cooperation over confrontation [ 211 ]. Of late, priority has been given instead to economic and military hegemony, despite recognition of the climate threat, and without consultation with young people or seeming consideration of their aspirations. Scientists can support an ecumenical perspective of our shared future by expanding international cooperation. Awareness of the gathering climate storm will grow this decade, so we must increase scientific understanding worldwide as needed for climate restoration.
Third, we must take action to reduce and reverse Earth’s energy imbalance. Highest priority is to phase down emissions, but it is no longer feasible to rapidly restore energy balance via only GHG emission reductions. Additional action is almost surely needed to prevent grievous escalation of climate impacts including lock-in of sea level rise that could destroy coastal cities world-wide. At least several years will be needed to define and gain acceptance of an approach for climate restoration. This effort should not deter action on mitigation of emissions; on the contrary, the concept of human intervention in climate is distasteful to many people, so support for GHG emission reductions will likely increase. Temporary solar radiation management (SRM) will probably be needed, e.g. via purposeful injection of atmospheric aerosols. Risks of such intervention must be defined, as well as risks of no intervention; thus, the U.S. National Academy of Sciences recommends research on SRM [ 212 ]. The Mt. Pinatubo eruption of 1991 is a natural experiment [ 213 , 214 ] with a forcing that reached [ 30 ] –3 W/m 2 . Pinatubo deserves a coordinated study with current models. The most innocuous aerosols may be fine salty droplets extracted from the ocean and sprayed into the air by autonomous sailboats [ 215 ]. This approach has been discussed for potential use on a global scale [ 216 ], but it needs research into potential unintended effects [ 217 ]. This decade may be our last chance to develop the knowledge, technical capability, and political will for actions needed to save global coastal regions from long-term inundation.
Politics and climate change
Actions needed to drive carbon intensity to zero—most important a rising carbon fee—are feasible, but not happening. The first author gained perspective on the reasons why during trips to Washington, DC, and to other nations at the invitation of governments, environmentalists, and, in one case, oil executives in London. Politicians from right (conservative) and left (progressive) parties are affected by fossil fuel interests. The right denies that fossil fuels cause climate change or says that the effect is exaggerated. The left takes up the climate cause but proposes actions with only modest effect, such as cap-and-trade with offsets, including giveaways to the fossil fuel industry. The left also points to work of Amory Lovins as showing that energy efficiency plus renewables (mainly wind and solar energy) are sufficient to phase out fossil fuels. Lovins says that nuclear power is not needed. It is no wonder that the President of Shell Oil would write a foreword with praise for Lovins’ book, Reinventing Fire [ 218 ], and that the oil executives in London did not see Lovins’ work as a threat to their business.
Opportunities for progress often occur in conjunction with crises. Today, the world faces a crisis—political polarization, especially in the United States—that threatens effective governance. Yet the crisis offers an opportunity for young people to help shape the future of the nation and the planet. Ideals professed by the United States at the end of World War II were consummated in formation of the United Nations, the World Bank, the Marshall Plan, and the Universal Declaration of Human Rights. Progress toward equal rights continued, albeit slowly. The ‘American dream’ of economic opportunity was real, as most people willing to work hard could afford college. Immigration policy welcomed the brightest; NASA in the 1960s invited scientists from European countries, Japan, China, India, Canada, and those wanting to stay found immigration to be straightforward. But the power of special interests in Washington grew, government became insular and inefficient, and Congress refused to police itself. Their first priority became reelection and maintenance of elite status, supported by special interests. Thousands of pages of giveaways to special interests lard every funding bill, including the climate bill titled ‘Inflation Reduction Act’—Orwellian double-speak—as the funding is borrowed from young people via deficit spending. The public is fed up with the Washington swamp but hamstrung by rigid two-party elections focused on a polarized cultural war.
A political party that takes no money from special interests is essential to address political polarization, which is necessary if the West is to be capable of helping preserve the planet and a bright future for coming generations. Young people showed their ability to drive an election—via their support of Barack Obama in 2008 and Bernie Sanders in 2016—without any funding from special interests. Groundwork is being laid to allow third party candidates in 2026 and 2028 elections in the U.S. Ranked voting is being advocated in every state to avoid the ‘spoiler’ effect of a third party. It is asking a lot to expect young people to grasp the situation that they have been handed—but a lot is at stake. As they realize that they are being handed a planet in decline, the first reaction may be to stamp their feet and demand that governments do better, but that has little effect. Nor is it sufficient to parrot big environmental organizations, which are now part of the problem, as they are partly supported by the fossil fuel industry and wealthy donors who are comfortable with the status quo. Instead, young people have the opportunity to provide the drive for a revolutionary third party that restores democratic ideals while developing the technical knowledge that is needed to navigate the stormy sea that their world is setting out upon.
We thank Eelco Rohling for inviting JEH to describe our perspective on global climate response to human-made forcing. JEH began to write a review of past work, but a paper on the LGM by Jessica Tierney et al . [ 49 ] and data on changing ship emissions provided by Leon Simons led to the need for new analyses and division of the paper into two parts. We thank Jessica also for helpful advice on other related research papers, Jim Zachos and Thomas Westerhold for explanations of their data and interpretations, Ed Dlugokencky of the NOAA Earth System Research Laboratory for continually updated GHG data, and David Arthur for pointing out the paper by Steinberger et al . JEH designed the study and carried out the research with help of Makiko Sato and Isabelle Sangha; Larissa Nazarenko provided data from GISS models and helped with analysis; Leon Simons provided ship emission information and aided interpretations; Pushker Kharecha provided critical review of the paper; James Zachos provided critical interpretation of ocean core data needed for interpretation of Cenozoic climate; Norman Loeb and Karina von Schuckmann provided EEI data and insight about implications; Matthew Osman provided paleoclimate data and an insightful review of an early draft paper; Qinjian Jin provided simulations of atmospheric sulfate and interpretations; Eunbi Jeong reviewed multiple drafts and advised on presentation; all authors contributed to our research summarized in the paper and reviewed and commented on the manuscript. Climate Science, Awareness and Solutions, which is directed by JEH and supports MS and PK is a 501(C3) nonprofit supported 100% by public donations. Principal supporters in the past few years have been the Grantham Foundation, Frank Batten, Eric Lemelson, James and Krisann Miller, Carl Page, Peter Joseph, Ian Cumming, Gary and Claire Russell, Donald and Jeanne Keith Ferris, Aleksandar Totic, Chris Arndt, Jeffrey Miller, Morris Bradley and about 150 more contributors to annual appeals.
Supplementary data are available at Oxford Open Climate Change online.
The authors declare that they have no conflict of interest.
The data used to create the Figs in this paper are available in the Zenodo repository, at https://zenodo.org/record/8419583 .
James Hansen (Conceptualization [lead], Data curation [equal], Formal analysis [lead], Funding acquisition [lead], Investigation [lead], Methodology [lead], Project administration [lead], Resources [lead], Software [equal], Supervision [lead], Validation [lead], Visualization [equal], Writing—original draft [lead], Writing—review and editing [lead]), Makiko Sato (Data curation [equal], Formal analysis [supporting], Investigation [supporting], Methodology [supporting], Project administration [supporting], Resources [supporting], Software [equal], Supervision [supporting], Validation [supporting], Visualization [equal], Writing—original draft [supporting], Writing—review and editing [supporting]), Leon Simons (Data curation [supporting], Formal analysis [supporting], Investigation [supporting], Methodology [supporting], Resources [supporting], Software [supporting], Supervision [supporting], Validation [supporting], Visualization [supporting], Writing—review and editing [supporting]), Larissa S. Nazarenko (Data curation [supporting], Formal analysis [supporting], Investigation [supporting], Methodology [supporting], Resources [supporting], Software [supporting], Supervision [supporting], Validation [supporting], Visualization [supporting], Writing—review and editing [supporting]), Isabelle Sangha (Formal analysis [supporting], Investigation [supporting], Methodology [supporting], Resources [supporting], Software [supporting], Supervision [supporting], Validation [supporting], Writing—review and editing [supporting]), Pushker Kharecha (Formal analysis [supporting], Investigation [supporting], Methodology [supporting], Resources [supporting], Software [supporting], Supervision [supporting], Validation [supporting], Writing—review and editing [supporting]), James Zachos (Data curation [supporting], Resources [supporting], Writing—review and editing [supporting]), Karina von Schuckmann (Formal analysis [supporting], Investigation [supporting], Methodology [supporting], Resources [supporting], Software [supporting], Supervision [supporting], Validation [supporting], Writing—review and editing [supporting]), Norman G. Loeb (Data curation [supporting], Formal analysis [supporting], Investigation [supporting], Methodology [supporting], Resources [supporting], Software [supporting], Supervision [supporting], Validation [supporting], Visualization [supporting], Writing—review and editing [supporting]), Matthew B. Osman (Data curation [supporting], Formal analysis [supporting], Investigation [supporting], Methodology [supporting], Resources [supporting], Software [supporting], Supervision [supporting], Validation [supporting], Visualization [supporting], Writing—review and editing [supporting)], Qinjian Jin (Data curation [supporting], Formal analysis [supporting], Investigation [supporting], Methodology [supporting], Resources [supporting], Software [supporting], Supervision [supporting], Validation [supporting], Visualization [supporting], Writing—review and editing [supporting]), George Tselioudis (Formal analysis [supporting], Investigation [supporting], Methodology [supporting], Resources [supporting], Software [supporting], Supervision [supporting], Writing—review and editing [supporting]), Eunbi Jeong (Formal analysis [supporting], Investigation [supporting], Methodology [supporting], Resources [supporting], Software [supporting], Supervision [supporting], Validation [supporting], Writing—review and editing [supporting]), Andrew Lacis (Formal analysis [supporting], Investigation [supporting], Methodology [supporting], Resources [supporting], Software [supporting], Supervision [supporting], Validation [supporting], Writing—review and editing [supporting]), Reto Ruedy (Formal analysis [supporting], Investigation [supporting], Methodology [supporting], Resources [supporting], Software [supporting], Supervision [supporting], Validation [supporting], Writing—review and editing [supporting]), Gary Russell (Formal analysis [supporting], Investigation [supporting], Methodology [supporting], Resources [supporting], Software [supporting], Supervision [supporting], Validation [supporting], Writing—review and editing [supporting]), Junji Cao (Formal analysis [supporting], Investigation [supporting], Methodology [supporting], Resources [supporting], Software [supporting], Supervision [supporting], Validation [supporting], Writing—review and editing [supporting]), Jing Li (Formal analysis [supporting], Investigation [supporting], Methodology [supporting], Resources [supporting], Software [supporting], Supervision [supporting], Validation [supporting], Writing—review and editing [supporting]).
Drafts of the chapters of Sophie’s Planet relevant to climate sensitivity are available here ; criticisms are welcome.
David EE, Jr later became a global warming denier.
GISS (2020) model is described as GISS-E2.1-G-NINT in published papers; NINT (noninteractive) signifies that the models use specified GHG and aerosol amounts.
An imbalance of 1 W/m 2 for a millennium is enough energy to melt ice raising sea level 110 m or to raise the temperature of the ocean’s upper kilometer by 11°C.
Tom Delworth (NOAA Geophysical Fluid Dynamics Laboratory), Gokhan Danabasoglu (National Center for Atmospheric Research), and Jonathan Gregory (UK Hadley Centre) kindly provided long 2 × CO 2 runs of GCMs of these leading modeling groups. All three models had response time as slow or slower than the GISS GCM.
The GISS (2014) model is labeled as GISS-E2-R-NINT and GISS (2020) as GISS-E2.1-G-NINT in published papers, where NINT (noninteractive) signifies that the models use specified GHG and aerosol amounts.
In Swedish, trapps are stairs. Basalt formations are commonly in layers from multiple extrusions.
Small apparent discrepancy is roundoff. CO 2 forcing is 9.13 W/m 2 and solar forcing is −1.16 W/m 2 at 50 MyBP.
Forcing = 4.6 W/m 2 assumes that the increase of non-CO 2 GHGs is human-made. This is true for CFCs and most trace gases, but a small part of CH 4 and N 2 O growth could be a slow feedback, slightly reducing the GHG forcing.
9.9°C for ECS = 1.2°C per W/m 2 ; 10.1°C for ECS = 1.22°C per W/m 2 (the precise ECS for 7°C LGM cooling).
Two significant flaws in the derivation of this ‘alternative aerosol scenario’ were largely offsetting: (1) the intermediate climate response function employed was too ‘fast’, but (2) this was compensated by use of a low climate sensitivity of 3°C for 2 × CO 2 .
In the absence of a response function from a GCM with ECS = 4°C, we use the normalized response function of the GISS (2020) model and put λ = 1°C per W/m 2 in Equation (5) .
Jay Zwally, Eric Rignot, Konrad Steffen, and Roger Braithwaite.
Tyndall J. On the absorption and radiation of heat by gases and vapours . Phil Mag 1861 ; 22 : 169 – 194 , 273 – 285 .
Google Scholar
Hansen J. Greenhouse Giants, Chapter 15 in Sophie’s Planet . New York : Bloomsbury , 2024 .
Google Preview
Revelle R , Broecker W , Craig H et al. Appendix Y4 atmospheric carbon dioxide. In: Hornig J, York HF, Branscomb LM et al. (eds,) President’s Science Advisory Committee. Restoring the Quality of Our Environment . Washington : The White House , 1965 , 111 – 33 .
Charney J , Arakawa A , Baker D et al. Carbon Dioxide and Climate: A Scientific Assessment . Washington : National Academy of Sciences Press , 1979 .
Nierenberg WA. Changing Climate: Report of the Carbon Dioxide Assessment Committee . Washington : National Academies Press , 1983 .
Hansen JE , Takahashi T (eds). AGU Geophysical Monograph 29 Climate Processes and Climate Sensitivity . Washington : American Geophysical Union , 1984 .
Hansen J , Lacis A , Rind D et al. Climate sensitivity: analysis of feedback mechanisms. In: Hansen JE, Takahashi T (eds), AGU Geophysical Monograph 29 Climate Processes and Climate Sensitivity . Washington : American Geophysical Union , 1984 , 130 – 63 .
David EE Jr . Inventing the future: energy and the CO 2 “Greenhouse Effect”. In: Hansen JE, Takahashi T (eds). AGU Geophysical Monograph 29 Climate Processes and Climate Sensitivity . Washington : American Geophysical Union , 1984 , 1 – 5 .
Oreskes N , Conway E. Merchants of Doubt: How a Handful of Scientists Obscured the Truth on Issues from Tobacco Smoke to Global Warming . London : Bloomsbury , 2010 .
Intergovernmental Panel on Climate Change . History of the IPCC . https://www.ipcc.ch/about/history (date last accessed 7 March 2023).
United Nations Framework Convention on Climate Change . What is the United Nations Framework Convention on Climate Change? https://unfccc.int/process-and-meetings/what-is-the-united-nations-framework-convention-on-climate-change ) (date last accessed 30 November 2022 ).
IPCC . Climate Change 2021: The Physical Science Basis . [Masson-Delmotte V, Zhai P, Pirani A et al. (eds)], Cambridge and New York : Cambridge University Press , 2021 .
Hansen J , Sato M , Hearty P et al. Ice melt, sea level rise and superstorms: evidence from paleoclimate data, climate modeling, and modern observations that 2 C global warming could be dangerous . Atmos Chem Phys 2016 ; 16 : 3761 – 812 .
Hansen J. Foreword: uncensored science is crucial for global conservation. In: DellaSala DA (ed), Conservation Science and Advocacy for a Planet in Peril . Amsterdam : Elsevier , 2021 , 451 .
Bode HW. Network Analysis and Feedback Amplifier Design . New York : Van Nostrand , 1945 .
Lacis A , Hansen J , Lee P et al. Greenhouse effect of trace gases, 1970-1980 . Geophys Res Lett 1981 ; 8 : 1035 – 8 .
CLIMAP Project Members . Seasonal reconstruction of the Earth’s surface at the last glacial maximum. Geol Soc Amer, Map and Chart Series , No. 36, Geological Society of America 1981 .
Manabe S , Stouffer RJ. Sensitivity of a global climate model to an increase of CO 2 concentration in the atmosphere . J Geophys Res 1980 ; 85 : 5529 – 54 .
Manabe S. Carbon dioxide and climate change . Adv Geophys 1983 ; 25 : 39 – 82 .
Klein SA , Hall A , Norris JR et al. Low-cloud feedbacks from cloud-controlling factors: a review . Surv Geophys 2017 ; 38 : 1307 – 29 .
Sherwood SC , Webb MJ , Annan JD et al. An assessment of Earth’s climate sensitivity using multiple lines of evidence . Rev Geophys 2020 ; 58 : e2019RG000678 .
Zelinka MD , Zhou C , Klein SA. Insights from a refined decomposition of cloud feedbacks . Geophys Res Lett 2016 ; 43 : 9259 – 69 .
Zelinka M , Tan I , Oreopoulos L et al. Detailing cloud property feedbacks with a regime-based decomposition . Clim Dyn 2023 ; 60 : 2983 – 3003 .
Rind D , Peteet D. Terrestrial conditions at the last glacial maximum and CLIMAP sea-surface temperature estimates: Are they consistent? Quat Res 1985 ; 24 : 1 – 22 .
Rohling EJ , Marino G , Foster GL et al. Comparing climate sensitivity, past and present . Ann Rev Mar Sci 2018 ; 10 : 261 – 88 .
IPCC . Climate Change 2014: Synthesis Report. Contribution of Working Groups I, II and III to the Fifth Assessment Report of the Intergovernmental Panel on Climate Change. [ Core Writing Team , Pachauri RK, Meyer LA (eds)]. Geneva : IPCC , 2014 .
Andrews T , Gregory JM , Paynter D et al. Accounting for changing temperature patterns increases historical estimates of climate sensitivity . Geophys Res Lett 2018 ; 45 : 8490 – 9 .
Rugenstein M , Bloch-Johnson J , Abe-Ouchi A et al. LongRunMIP: motivation and design for a large collection of millennial-length AOGCM simulations . Bull Amer Meteorol Soc 2019 ; 100 : 2551 – 70 .
Myhre G , Shindell D , Bréon F-M et al. Anthropogenic and natural radiative forcing. In: Stocker TF, Qin D, Plattner G-K et al. (eds), Climate Change 2013: The Physical Science Basis. Contribution of Working Group I to the Fifth Assessment Report of the Intergovernmental Panel on Climate Change . Cambridge and New York : Cambridge University Press , 2013 .
Hansen J , Sato M , Ruedy R et al. Efficacy of climate forcings . J Geophys Res 2005 ; 110 : D18104 .
Lohmann U , Rotstayn L , Storelvmo T et al. Total aerosol effect: radiative forcing or radiative flux perturbation? Atmos Chem Phys 2010 ; 10 : 3235 – 46 .
Kelley M , Schmidt GA , Nazarenko L et al. GISS-E2.1: configurations and climatology . J Adv Model Earth Syst 2020 ; 12 : e2019MS002025 .
Miller RL , Schmidt GA , Nazarenko L et al. CMIP6 historical simulations (1850-2014) with GISS-E2.1 . J Adv Model Earth Syst 2021 ; 13 : e2019MS002034 .
Eyring V , Bony S , Meehl GA et al. Overview of the Coupled Model Intercomparison Project Phase 6 (CMIP6) experimental design and organization . Geosci Model Dev 2016 ; 9 : 1937 – 58 .
Lacis AA , Oinas V. A description of the correlated k distributed method for modeling nongray gaseous absorption, thermal emission, and multiple scattering in vertically inhomogeneous atmospheres . J Geophys Res 1991 ; 96 : 9027 – 63 .
Rothman L , Rinsland C , Goldman A et al. The HITRAN molecular spectroscopic database and HAWKS (HITRAN Atmospheric Workshation) 1996 edition . J Quan Spec Rad Trans 1998 ; 60 : 665 – 710 .
Prather M , Ehhalt D. Chapter 4: Atmospheric chemistry and greenhouse gases. In: Houghton JT (ed), Climate Change 2001: The Scientific Basis . New York : Cambridge University , 2001 , 239 – 87 .
Hansen J , Sato M. Greenhouse gas growth rates . Proc Natl Acad Sci U S A 2004 ; 101 : 16109 – 14 .
Columbia University . MPTG and OTG data : www.columbia.edu/~mhs119/GHGs/TG_F.1900-1990.txt and http://www.columbia.edu/~mhs119/GHGs/TG_F.1992-2021.txt (date last accessed 9 August 2023).
Jouzel J , Masson-Delmotte V , Cattani O et al. Orbital and millennial Antarctic climate variability over the past 800,000 years . Science 2007 ; 317 : 793 – 6 .
Luthi D , Le Floch M , Bereiter B et al. High-resolution carbon dioxide concentration record 650,000-800,000 years before present . Nature 2008 ; 453 : 379 – 82 .
Hays JD , Imbrie J , Shackleton NJ. Variation in the Earth’s orbit: pacemaker of the ice ages . Science 1976 ; 194 : 1121 – 32 .
Lorius C , Jouzel J , Raynaud D et al. The ice-core record: climate sensitivity and future greenhouse warming . Nature 1990 ; 347 : 139 – 45 .
Zachos J , Pagani M , Sloan L et al. Trends, rhythms, and aberrations in global climate 65 Ma to present . Science 2001 ; 292 : 686 – 93 .
Hansen J , Sato M , Kharecha P et al. Climate change and trace gases . Philos Trans A Math Phys Eng Sci 2007 ; 365 : 1925 – 54 .
Ruddiman WF , Fuller DQ , Kutzbach JE et al. Late Holocene climate: natural or anthropogenic? Rev Geophys 2016 ; 54 : 93 – 118 .
Schilt A , Baumgartner M , Schwander J et al. Atmospheric nitrous oxide during the last 140,000 years . Earth Planet Sci Lett 2010 ; 300 : 33 – 43 .
Hansen J , Nazarenko L , Ruedy R et al. Earth's energy imbalance: Confirmation and implications . Science 2005 ; 308 : 1431 – 5 .
Tierney JE , Zhu J , King J et al. Glacial cooling and climate sensitivity revisited . Nature 2020 ; 584 : 569 – 73 .
Osman MB , Tierney JE , Zhu J et al. Globally resolved surface temperatures since the Last Glacial Maximum . Nature 2021 ; 599 : 239 – 44 .
Seltzer AM , Ng J , Aeschbach W et al. Widespread six degrees Celsius cooling on land during the Last Glacial Maximum . Nature 2021 ; 593 : 228 – 32 .
Schneider T , Teixeira J , Bretherton CS et al. Climate goals and computing the future of clouds . Nature Clim Change 2017 ; 7 : 3 – 5 .
Pincus R , Forster PM , Stevens B. The radiative forcing model intercomparison project (RFMIP): experimental protocol for CMIP6 . Geosci Model Dev 2016 ; 9 : 3447 – 60 .
Kageyama M , Braconnot P , Harrison SP et al. The PMIP4 contribution to CMIP6 – Part 1: overview and over-arching analysis plan . Geosci Model Dev 2018 ; 11 : 1033 – 57 .
Hegerl GC , Zwiers FW , Braconnot P et al. Chapter 9: Understanding and attributing climate change. In: Solomon SD (ed), Climate Change 2007: The Physical Science Basis . New York : Cambridge University , 2007 , 663 – 745
Yoshimori M , Yokohata T , Abe-Ouchi A. A comparison of climate feedback strength between CO 2 doubling and LGM experiments . J Clim 2009 ; 22 : 3374 – 95 .
Stap LB , Kohler P , Lohmann G. Including the efficacy of land ice changes in deriving climate sensitivity from paleodata . Earth Syst Dynam 2019 ; 10 : 333 – 45 .
Koppen W. Das geographische system der climate. In: Koppen W, Geiger G (eds), Handbuch Der Klimatologie 1(C). Berlin : Boentraeger , 1936 .
Kohler P , Bintanja R , Fischer H et al. What caused Earth’s temperature variations during the last 800,000 years? Data-based evidence on radiative forcing and constraints on climate sensitivity . Quat Sci Rev 2010 ; 29 : 129 – 45 .
Hansen J , Sato M , Kharecha P et al. Target atmospheric CO 2 : where should humanity aim? Open Atmos Sci J 2008 ; 2 : 217 – 31 .
Rabineau M , Berné S , Olivet J-L et al. Paleo sea levels reconsidered from direct observation of paleoshoreline position during Glacial Maxima (for the last 500,000 yr) . Earth Planet Sci Lett 2006 ; 252 : 119 – 37 .
Rohling EJ , Hibbert FD , Williams FH et al. Differences between the last two glacial maxima and implications for ice-sheet, ō18O, and sea-level reconstructions . Quat Sci Rev 2017 ; 176 : 1 – 28 .
Hansen J , Sato M , Kharecha P et al. Young people’s burden: requirement of negative CO2 emissions . Earth Syst Dynam 2017 ; 8 : 577 – 616 .
Hoffman JS , Clark PU , Parnell AC et al. Regional and global sea-surface temperatures during the last interglaciation . Science 2017 ; 355 : 276 – 9 .
Ruth U , Barnola JM , Beer J et al. EDML1: a chronology for the EPICA deep ice core from Dronning Maud Land, Antarctica, over the last 150 000 years . Clim Past 2007 ; 3 : 475 – 84 .
Hansen J , Sato M , Russell G et al. Climate sensitivity, sea level, and atmospheric carbon dioxide . Philos Trans A Math Phys Eng Sci 2013 ; 371 : 20120294 .
Russell GL , Miller JR , Rind D. A coupled atmosphere-ocean model for transient climate change studies . Atmos Ocean 1995 ; 33 : 683 – 730 .
Hoffman PF , Schrag DP. The snowball Earth hypothesis: testing the limits of global change . Terra Nova 2002 ; 14 : 129 – 55 .
Sackmann J , Boothroyd AI , Kraemer KE. Our Sun. III. Present and future . Astrophys J 1993 ; 418 : 457 – 68 .
Meraner K , Mauritsen T , Voigt A. Robust increase in equilibrium climate sensitivity under global warming . Geophys Res Lett 2013 ; 40 : 5944 – 8 .
Lunt DJ , Haywood AM , Schmidt GA et al. Earth system sensitivity inferred from Pliocene modelling and data . Nature Geosci 2010 ; 3 : 60 – 4 .
Beerling DJ , Fox A , Stevenson DS et al. Enhanced chemistry-climate feedbacks in past greenhouse worlds . Proc Natl Acad Sci U S A 2011 ; 108 : 9770 – 5 .
Bryan K , Komro FG , Manabe S et al. Transient climate response to increasing atmospheric carbon dioxide . Science 1982 ; 215 : 56 – 8 .
Hansen J , Russell G , Lacis A et al. Climate response times: dependence on climate sensitivity and ocean mixing . Science 1985 ; 229 : 857 – 9 .
Hansen J. Climate Threat to the Planet, American Geophysical Union , San Francisco, California, 17 December 2008 . http://www.columbia.edu/~jeh1/2008/AGUBjerknes20081217.pdf . (date last accessed 3 December 2022).
Good P , Gregory JM , Lowe JA. A step-response simple climate model to reconstruct and interpret AOGCM projections . Geophys Res Lett 2011 ; 38 : e2010GL0452008 .
Schmidt GA , Kelley M , Nazarenko L et al. Configuration and assessment of the GISS ModelE2 contributions to the CMIP5 archive . J Adv Model Earth Syst 2014 ; 6 : 141 – 84 .
Prather MJ. Numerical advection by conservation of second order moments . J Geophys Res 1986 ; 91 : 6671 – 81 .
Romanou A , Marshall J , Kelley M et al. Role of the ocean’s AMOC in setting the uptake efficiency of transient tracers . Geophys Res Lett 2017 ; 44 : 5590 – 8 .
von Schuckmann K , Cheng L , Palmer MD et al. Heat stored in the Earth system: where does the energy go? Earth Syst Sci Data 2020 ; 12 : 2013 – 41 .
Loeb NG , Johnson GC , Thorsen TJ et al. Satellite and ocean data reveal marked increase in Earth’s heating rate . Geophys Res Lett 2021 ; 48 : e2021GL093047 .
Hansen J , Johnson D , Lacis A et al. Climate impact of increasing atmospheric carbon dioxide . Science 1981 ; 213 : 957 – 66 .
Kamae Y , Watanabe M , Ogura T et al. Rapid adjustments of cloud and hydrological cycle to increasing CO 2 : a review . Curr Clim Change Rep 2015 ; 1 : 103 – 13 .
Zelinka MD , Myers TA , McCoy DT et al. Causes of higher climate sensitivity in CMIP6 models . Geophys Res Lett 2020 ; 47 : e2019GL085782 .
Crowley TJ. Pliocene climates: the nature of the problem . Marine Micropaleontol 1996 ; 27 : 3 – 12 .
DeConto RM , Pollard D. Rapid Cenozoic glaciation of Antarctica induced by declining atmospheric CO 2 . Nature 2003 ; 421 : 245 – 9 .
Lacis AA , Schmidt GA , Rind D et al. Atmospheric CO 2 : principal control knob governing Earth’s temperature . Science 2010 ; 330 : 356 – 9 .
Rae JWB , Zhang YG , Liu X et al. Atmospheric CO 2 over the past 66 million years from marine archives . Annu Rev Earth Planet Sci 2021 ; 49 : 609 – 41 .
Steinthorsdottir M , Vajda V , Pole M et al. Moderate levels of Eocene pCO 2 indicated by Southern Hemisphere fossil plant stomata . Geology 2019 ; 47 : 914 – 8 .
Westerhold T , Marwan N , Drury AJ et al. An astronomically dated record of Earth’s climate and its predictability over the last 66 million years . Science 2020 ; 369 : 1383 – 7 .
Yatheesh V , Dyment J , Bhattacharya GC et al. Detailed structure and plate reconstructions of the central Indian Ocean between 83.0 and 42.5 Ma (chrons 34 and 20) . J Geophys Res: Solid Earth 2019 ; 124 : 4305 – 22 .
Cutler KB , Edwards RL , Taylor FW et al. Rapid sea-level fall and deep-ocean temperature change since the last interglacial period . Earth Planet Sci Lett 2003 ; 206 : 253 – 71 .
Siddall M , Honisch B , Waelbroeck C et al. Changes in deep Pacific temperature during the mid-Pleistocene transition and Quaternary . Quatern Sci Rev 2010 ; 29 : 170 – 81 .
Rohling EJ , Grant K , Bolshaw M et al. Antarctic temperature and global sea level closely coupled over the past five glacial cycles . Nature Geosci 2009 ; 2 : 500 – 4 .
Seltzer AM , Blard P-H , Sherwood SC et al. Terrestrial amplification of past, present, and future climate change . Sci Adv 2023 ; 9 : eadf8119 .
Zhu J , Poulsen CJ , Tierney JE. Simulation of Eocene extreme warmth and high climate sensitivity through cloud feedbacks . Sci Adv 2019 ; 5 : eaax1874 .
Scotese C. PALEOMAP PaleoAtlas for GPlates , 2016. https://www.earthbyte.org/paleomap-paleoatlas-for-gplates/ (March 2023, date last accessed).
Hansen J. Storms of My Grandchildren . New York : Bloomsbury , 2009 .
Berner RA. The Phanerozoic Carbon Cycle: CO2 and O2 . New York : Oxford University Press , 2004 .
Rohling EJ. The Climate Question: natural Cycles, Human Impact, Future Outlook . New York: Oxford University Press , 2019 .
Merdith AS , Williams SE , Brune S et al. Rift and plate boundary evolution across two supercontinent cycles . Global Plan Chan 2019 ; 173 : 1 – 14 .
Peace AL , Phethean JJJ , Franke D et al. A review of Pangea dispersal and large igneous provinces – in search of a causative mechanism . Earth-Science Rev 2020 ; 206 : 102902 .
Baksi AK. Comment on “40Ar/39Ar dating of the Rajahmundry Traps, eastern India and their relationship to the Deccan Traps” by Knight et al. [Earth Planet Sci. Lett. 208 (2003) 85-99] . Earth Planet Sci Lett 2005 ; 239 : 368 – 73 .
Guo Z , Wilson M , Dingwell D et al. India-Asia collision as a driver of atmospheric CO 2 in the Cenozoic . Nature Comm 2021 ; 12 : 3891 .
Raymo ME , Ruddiman WF. Tectonic forcing of late Cenozoic climate . Nature 1992 ; 359 : 117 – 22 .
Ramos EJ , Lackey JS , Barnes JD et al. Remnants and rates of metamorphic decarbonation in continental arcs . Gsat 2020 ; 30 : 4 – 10 .
Bufe A , Hovius N , Emberson R et al. Co-variation of silicate, carbonate and sulfide weathering drives CO 2 release with erosion . Nat Geosci 2021 ; 14 : 211 – 6 .
Lee CTA , Shen B , Slotnick BS et al. Continental arc-island arc fluctuations, growth of crustal carbonates, and long-term climate change . Geosphere 2013 ; 9 : 21 – 36 .
McKenzie NR , Horton BK , Loomis SE et al. Continental arc volcanism as the principal driver of icehouse-greenhouse variability . Science 2016 ; 352 : 444 – 7 .
Petersen KD , Schiffer C , Nagel T. LIP formation and protracted lower mantle upwelling induced by rifling and delamination . Scientific Rep 2018 ; 8 : 16578 .
Eldholm E , Grue K. North Atlantic volcanic margins: dimensions and production rates . J Geophys Res 1994 ; 99 : 2955 – 68 .
Ji S , Nie J , Lechler A et al. A symmetrical CO 2 peak and asymmetrical climate change during the middle Miocene . Earth Plan Sci Lett 2018 ; 499 : 134 – 44 .
Babila TL , Foster GL. The Monterey Event and the Paleocene-Eocene Thermal Maximum: two contrasting oceanic carbonate system responses to LIP emplacement and eruption. In: Dickson A, Bekker A (eds), AGU Geographical Monograph 255, Washington: American Geophysical Union, 2021, 403 – 416 .
Storey M , Duncan RA , Tegner C. Timing and duration of volcanism in the North Atlantic Igneous Province: implications for geodynamics and links to the Iceland hotspot . Chem Geol 2007 ; 241 : 264 – 81 .
Svensen H , Planke S , Malthe-Sorenssen A et al. Release of methane from a volcanic basin as a mechanism for initial Eocene global warming . Nature 2004 ; 429 : 542 – 5 .
Gutjahr M , Ridgwell A , Sexton PF et al. Very large release of mostly volcanic carbon during the Palaeocene Thermal Maximum . Nature 2017 ; 548 : 573 – 7 .
Frieling J , Peterse F , Lunt DJ et al. Widespread warming before and elevated barium burial during the Paleocene-Eocene thermal maximum: evidence for methane hydrate release? Paleoceanogr Paleoclimatol 2019 ; 34 : 546 – 66 .
Berndt C , Planke S , Alvarez Zarikian CA et al. Shallow-water hydrothermal venting linked to the Palaeocene-Eocene Thermal Maximum . Nature Geosci 2023 ; 16 : 803 – 9 .
Walker JCG , Hays PB , Kasting JF. A negative feedback mechanism for the long-term stabilization of Earth’s surface temperature . J Geophys Res 1981 ; 86 : 9776 – 82 .
Steinberger B , Spakman W , Japsen P et al. The key role of global solid-Earth processes in preconditioning Greenland’s glaciation since the Pliocene . Terra Nova 2015 ; 27 : 1 – 8 .
Foster GL , Hull P , Lunt DJ et al. Placing our current ‘hyperthermal’ in the context of rapid climate change in our geological past . Phil Trans Roy Soc A 2018 ; 376 : 200170086 .
Tierney JE , Zhu J , Li M. Spatial patterns of climate change across the Paleocene-Eocene thermal maximum . Proc Natl Acad Sci 2022 ; 119 : e2205326119 .
Hopcroft PO , Ramstein G , Pugh TAM et al. Polar amplification of Pliocene climate by elevated trace gas radiative forcing . Proc Natl Acad Sci U S A 2020 ; 117 : 23401 – 7 .
Schaller MF , Fung MK. The extraterrestrial impact evidence at the Palaeocene-Eocene boundary and sequence of environmental change on the continental shelf . Phil Trans R Soc A 2018 ; 376 : 20170081 .
Turner SK. Constraints on the onset duration of the Paleocene-Eocene Thermal Maximum . Phil Trans R Soc A 2018 ; 376 : 20170082 .
Zachos JC , McCarren H , Murphy B et al. Tempo and scale of late Paleocene and early Eocene carbon isotope cycles: implications for the origin of hyperthermals . Earth Plan Sci Lett 2010 ; 299 : 242 – 9 .
Nichols JE , Peteet DM. Rapid expansion of northern peatlands and doubled estimate of carbon storage . Nat Geosci 2019 ; 12 : 917 – 21 .
Hanson PJ , Griffiths NA , Iverson CM et al. Rapid net carbon loss from a whole-ecosystem warmed peatland . AGU Advan 2020 ; 1 : e2020AV000163 .
Bowen GJ , Maibauer BJ , Kraus MJ et al. Two massive, rapid releases of carbon during the onset of the Palaeocene-Eocene thermal maximum . Nature Geosci 2015 ; 8 : 44 – 7 .
Archer D , Buffett B , Brovkin V. Ocean methane hydrates as a slow tipping point in the global carbon cycle . Proc Natl Acad Sci U S A 2009 ; 106 : 20596 – 601 .
Archer D , Eby M , Brovkin V et al. Atmospheric lifetime of fossil fuel carbon dioxide . Annu Rev Earth Planet Sci 2009 ; 37 : 117 – 34 .
Nunes F , Norris RD. Abrupt reversal in ocean overturning during the Palaeocene/Eocene warm period . Nature 2006 ; 439 : 60 – 3 .
World Health Organization . Ambient (Outdoor) Air Pollution . https://www.who.int/en/news-room/fact-sheets/detail/ambient-(outdoor)-air-quality-and-health (date last accessed 23 June 2022).
Vohra K , Vodonos A , Schwartz J et al. Global mortality from outdoor fine particle pollution generated by fossil fuel combustion: results from GEOS-Chem . Environ Res 2021 ; 195 : 110754 .
Marcott SA , Shakun JD , Clark PU et al. A reconstruction of regional and global temperature for the last 11,300 . Science 2013 ; 339 : 1198 – 201 .
Tardif R , Hakim GJ , Perkins WA et al. Last Millenium Reanalysis with an expanded proxy database and seasonal proxy modeling . Clim Past 2019 ; 15 : 1251 – 73 .
Watson AJ , Garabato ACN. The role of Southern Ocean mixing and upwelling in glacial-interglacial atmospheric CO 2 change . Tellus 2006 ; 58 : 73 – 87 .
Wikipedia . File:Post-Glacial Sea Level.png. https://commons.wikimedia.org/wiki/File:Post-Glacial_Sea_Level.png (date last accessed 3 December 2022).
Barber B. Resistance by scientists to scientific discovery . Science 1961 ; 134 : 596 – 602 .
Hoffman PF , Kaufman AJ , Halverson GP et al. A Neoproterozoic Snowball Earth . Science 1998 ; 281 : 1342 – 6 .
Alvarez L , Alvarez W , Asaro F et al. Extraterrestrial Cause for the Cretaceous-Tertiary Extinction . Science 1980 ; 208 : 1095 – 108 .
Mishchenko MI , Cairns B , Kopp G et al. Accurate monitoring of terrestrial aerosols and total solar irradiance: Introducing the Glory mission . Bull Amer Meteorol Soc 2007 ; 88 : 677 – 92 .
Hansen J , Rossow W , Fung I. Long-Term Monitoring of Global Climate Forcings and Feedbacks . Washington : NASA Conference Publication 3234 , 1993 .
Bellouin N , Quaas J , Gryspeerdt E et al. Bounding global aerosol radiative forcing of climate change . Rev Geophys 2020 ; 58 : e2019RG000660 .
Kruzman D. Wood-burning stoves raise new health concerns. Undark Magazine 2022 , 2 March (6 February 2023, date last accessed).
Glojek K , Mocnik G , Alas HDC et al. The impact of temperature inversions on black carbon and particle mass concentrations in a mountainous area . Atmos Chem Phys 2022 ; 22 : 5577 – 601 .
Rutgard O. Why is Britain Taking the Axe to Wood-Burning Stoves? Bloomberg Green , 4 February 2023 .
Day JW , Gunn JD , Folan WJ et al. Emergence of complex societies after sea level stabilized . EOS Transactions 2007 ; 88 : 169 – 70 .
VanCuren RA. Asian aerosols in North America: extracting the chemical composition and mass concentration of the Asian continental aerosol plume from long-term aerosol records in the western United States . J Geophs Res Atmos 2003 ; 108 : D20, 4623 .
Knutti R. Why are climate models reproducing the observed global surface warming so well? Geophys Res Lett 2008 ; 35 : L18704 .
Hansen J , Sato M , Kharecha P et al. Earth's energy imbalance and implications . Atmos Chem Phys 2011 ; 11 : 13421 – 49 .
Koch D , Bauer SE , Del Genio A et al. Coupled aerosol-chemistry-climate twentieth-century model investigation: trends in short-lived species and climate responses . J Clim 2011 ; 24 : 2693 – 714 .
Novakov T , Ramanathan V , Hansen JE et al. Large historical changes of fossil-fuel black carbon aerosols . Geophys Res Lett 2003 ; 30 : 1324 .
Bauer SE , Tsigaridis K , Faluvegi G et al. Historical (1850-2014) aerosol evolution and role on climate forcing using the GISS ModelE2.1 contribution to CMIP6 . J Adv Model Earth Syst 2020 ; 12 : e2019MS001978 .
Hansen J , Ruedy R , Sato M et al. Global surface temperature change . Rev Geophys 2010 ; 48 : RG4004 .
Lenssen NJL , Schmidt GA , Hansen JE et al. Improvements in the GISTEMP uncertainty model . JGR Atmospheres 2019 ; 124 : 6307 – 26 .
Jin Q , Grandey BS , Rothenberg D et al. Impacts on cloud radiative effects induced by coexisting aerosols converted from international shipping and maritime DMS emissions . Atmos Chem Phys 2018 ; 18 : 16793 – 808 .
Hansen J , Rossow W , Carlson B et al. Low-cost long-term monitoring of global climate forcings and feedbacks . Clim Chan 1995 ; 31 : 247 – 71 .
Glassmeier F , Hoffmann F , Johnson JS et al. Aerosol-cloud-climate cooling overestimated by ship-track data . Science 2021 ; 371 : 485 – 9 .
Manshausen P , Watson-Parris D , Christensen MW et al. Invisible ship tracks show large cloud sensitivity to aerosol . Nature 2022 ; 610 : 101 – 6 .
Wall CJ , Norris JR , Possner A et al. Assessing effective radiative forcing from aerosol-cloud interactions over the global ocean . Proc Natl Acad Sci U S A 2022 ; 119 : e2210481119 .
Forster P , Storelvmo T , Armour K et al. The Earth’s energy budget, climate feedbacks, and climate sensitivity. In: Masson-Delmotte V (ed), Climate Change 2021: The Physical Science Basis . New York, Cambridge : Cambridge University Press , 2021 , 923 – 1054 .
International Maritime Organization (IMO), MEPC.176(58) . Amendments to the annex of the protocol of 1997 to amend the international convention for the prevention of pollution from ships, 1973, as modified by the protocol of 1978 relating thereto (Revised MARPOL, Annex VI), 2008 . https://wwwcdn.imo.org/localresources/en/OurWork/Environment/Documents/176(58).pdf (February 2013, date last accessed).
Gryspeerdt E , Smith TWP , O'Keeffe E et al. The impact of ship emission controls recorded by cloud properties . Geophys Res Lett 2019 ; 46 : 547 – 55 .
International Maritime Organization . IMO 2020–Cutting Sulphur Oxide Emissions, Lowers Limit on Sulfur Content of Marine Fuels from 3.5% to 0.5%. https://www.imo.org/en/MediaCentre/HotTopics/Pages/Sulphur-2020.aspx (date last accessed 5 December 2022).
Yuan T , Song H , Wood R et al. Global reduction in ship-tracks from sulfur regulations for shipping fuel . Sci Adv 2022 ; 8 : eabn7988 .
Columbia University. Data sources, graphs . http://www.columbia.edu/~mhs119/Solar/ (date last accessed 23 October 2022).
Loeb NG , Thorsen TJ , Rose FG et al. Recent variations in EEI, SST & clouds. ERB Workshop, Hamburg, Germany, 12–14 October, 2022 (date last accessed 3 December 2022).
Sato M. Sea ice area. Columbia University webpage (date last accessed 5 November 2022 ).
McCoy DT , Burrows SM , Wood R et al. Natural aerosols explain seasonal and spatial patterns of Southern Ocean cloud albedo . Sci Adv 2015 ; 1 : e1500157 .
Dunne JP , Winton M , Bacmeister J et al. Comparison of equilibrium climate sensitivity estimates from slab ocean, 150‐year, and longer simulations . Geo Res Lett 2020 ; 47 : e2020GL088852 .
Forster PM , Maycock AC , McKenna CM et al. Latest climate models confirm need for urgent mitigation . Nat Clim Chang 2020 ; 10 : 7 – 10 .
Liu Z , Zhu J , Rosenthal Y et al. The Holocene temperature conundrum . Proc Natl Acad Sci U S A 2014 ; 111 : E3501 – E3505 .
Quaas J , Jia H , Smith C et al. Robust evidence for reversal of the trend in aerosol effective climate forcing . Atmos Chem Phys 2022 ; 22 : 12221 – 39 .
Bauer SE , Tsigaridis K , Faluvegi G et al. The turning point of the aerosol era . J Adv Mod Earth Syst 2022 ; 14 : e2022MS003070 .
Diamond MS. Detection of large-scale cloud microphysical changes and evidence for decreasing cloud brightness within a major shipping corridor after implementation of the International Maritime Organization 2020 fuel sulfur regulations . Atmos Chem Phys 2023 ; 23 : 8259 – 69 .
Hefner M , Marland G , Boden T et al. Global, Regional, and National Fossil-Fuel CO 2 Emissions . Research Institute for Environment, Energy, and Economics, Appalachian State University, Boone, NC, USA. https://energy.appstate.edu/cdiac-appstate/data-products (date last accessed 20 August 2023).
Energy Institute . 2023 Statistical Review of World Energy (date last accessed 20 August 2023 ).
Hansen J , Sato M , Ruedy R et al. Global warming in the twenty-first century: an alternative scenario . Proc Natl Acad Sci U S A 2000 ; 97 : 9875 – 80 .
Hansen JE (ed.). Air Pollution as a Climate Forcing: A Workshop . NASA Goddard Institute for Space Studies , 2002 .
Feynman RP. Surely You’re Joking, Mr. Feynman! . New York : WW Norton , 1985 .
Hariri AR , Brown SM , Williamson DE et al. Preference for immediate over delayed rewards is associated with magnitude of ventral striatal activity . J Neurosci 2006 ; 26 : 13213 – 7 .
Hansen JE. Scientific reticence and sea level rise . Environ Res Lett 2007 ; 2 : 024002 . er1246875
Hansen JE. A slippery slope: how much global warming constitutes “dangerous anthropogenic interference?” . Clim Change 2005 ; 68 : 269 – 79 .
Braithewaite RJ. Cover photo for Science 2002;297(5579). Reprinted in Hansen, J. Defusing the global warming time bomb . Sci Amer 2004 ; 290 : 68 – 77 .
Rignot E , Jacobs S , Mouginot J et al. Ice shelf melting around Antarctica . Science 2013 ; 341 : 266 – 70 .
Rye CD , Naveira Garabato AC , Holland PR et al. Rapid sea-level rise along the Antarctic margins in response to increased glacial discharge . Nature Geosci 2014 ; 7 : 732 – 5 .
Hansen J Sato M Hearty P et al. Ice Melt, Sea Level Rise and Superstorms: Evidence from Paleoclimate Data, Climate Modeling, and Modern Observations that 2 C Global Warming is Highly Dangerous. Atmospheric Chemistry and Physics Discussions. 2015.
Bakker P , Schmittner A , Lenaerts JTM et al. Fate of the Atlantic Meridional Overturning Circulation: strong decline under continued warming and Greenland melting . Geophy Res Lett 2016 ; 43 : 12252 – 60 .
Irvali N , Ninnemann US , Galaasen EV et al. Rapid switches in subpolar hydrography and climate during the Last Interglacial (MIS 5e) . Paleoceanography 2012 ; 27 :PA2207.
Blanchon P , Eisenhauer A , Fietzke J et al. Rapid sea-level rise and reef back-stepping at the close of the last interglacial highstand . Nature 2009 ; 458 : 881 – 4 .
Rye C , Marshall J , Kelley M et al. Antarctic glacial melt as a driver of recent Southern Ocean climate trends . Geophys Res Lett 2020 ; 47 :e2019GL086892.
Silvano A , Rintoul SR , Pena-Molino B et al. Freshening by glacial meltwater enhances melting of ice shelves and reduces formation of Antarctic bottom water . Sci Adv 2018 ; 4 : eaap9467 .
Gunn KL , Rintoul SR , England MH et al. Recent reduced abyssal overturning and ventilation in the Australian Antarctic Basin . Nat Clim Chang 2023 ; 13 : 537 – 44 .
Ditlevsen P , Ditlevsen S. Warning of a forthcoming collapse of the Atlantic meridional overturning circulation . Nature Comm 2023 ; 14 : 4254 .
Rignot E , Mouginot J , Scheuchl B et al. Four decades of Antarctic ice shelf mass balance from 1979-2017 . Proc Natl Acad Sci U S A 2018 ; 116 : 1096 – 103 .
Otosaka IN , Shepherd A , Ivins ER et al. Mass balance of the Greenland and Antarctic ice sheets from 1992 to 2020 . Earth Syst Sci Data 2023 ; 15 : 1597 – 616 .
Hansen J , Sato M , Ruedy R et al. Dangerous human-made interference with climate: A GISS modelE study . Atmos Chem Phys 2007 ; 7 : 2287 – 312 .
Matthews HD , Gillett NP , Stott PA et al. The proportionality of global warming to cumulative carbon emissions . Nature 2009 ; 459 : 829 – 32 .
Hansen J , Sato M. Regional Climate Change and National Responsibilities . Environ Res Lett 2016 ; 11 : 034009 .
Paris Agreement 2015 . UNFCCC Secretariat, 2015 . https://www.google.com/aclk?sa=l&ai=DChcSEwjrgqiAkf2BAxUz2EwCHfzPDPcYABABGgJ0bQ&gclid=Cj0KCQjw4bipBhCyARIsAFsieCzU7epQQG4ouyspwn7TPYa7IWh2W0OqZJVXr0qbGhLzshwOnCk1YEkaAvzLEALw_wcB&sig=AOD64_3vxA5GilCwgEGMqI4OhPZk0EBbbQ&q&adurl&ved=2ahUKEwja_52Akf2BAxVWsFYBHcL6CYcQ0Qx6BAgJEAE (date last accessed 20 August 2023).
Keith DW , Holmes G , Angelo D et al. A process for capturing CO 2 from the atmosphere . Joule 2018 ; 2 : 1573 – 94 .
Hansen J , Kharecha P. Cost of carbon capture: can young people bear the burden? Joule 2018 ; 2 : 1405 – 7 .
Hardin G. The tragedy of the commons . Science 1968 ; 162 : 1243 – 8 .
Prins G , Rayner S. Time to ditch Kyoto . Nature 2007 ; 449 : 973 – 5 .
Creutzig F , Erb KH , Haberl H et al. Considering sustainability thresholds for BECCS in IPCC and biodiversity assessments . GCB Bioemergy 2021 ; 13 : 510 – 5 .
Economists’ Statement on Carbon Dividends . https://www.historyismade.org/ (date last accessed 28 November 2022).
Hansen J. Can Young People Save Democracy and the Planet? https://www.columbia.edu/~jeh1/mailings/2021/20211008_YoungPeople.2021.pdf (date last accessed 28 November 2022)
Hayes RB. Nuclear energy myths versus facts support it’s expanded use – a review . Cleaner Ener Sys 2022 ; 2 : 100009 .
Cao J , Cohen A , Hansen J et al. China-U.S. cooperation to advance nuclear power . Science 2016 ; 353 : 547 – 8 .
Ying F. Cooperative competition is possible between China and the U.S. New York Times , 24 November.
National Academies of Sciences, Engineering, and Medicine . Reflecting Sunlight: Recommendations for Solar Geoengineering Research and Research Governance. https://doi.org/10.17226/25762 (date last accessed 4 December 2022).
Robock A. Volcanic eruptions and climate . Rev Geophys 2000 ; 38 : 191 – 219 .
Hansen J , Sato M , Ruedy R et al. A Pinatubo climate Modeling investigation . NATO ASI 1996 ; I42 : 234 – 72 .
Tollefson J. Can artificially altered clouds save the Great Barrier Reef? Nature 2021 ; 596 : 476 – 8 .
Latham J , Rasch P , Chen CC et al. Global temperature stabilization via controlled albedo enhancement of low-level maritime clouds . Philos Trans A Math Phys Eng Sci 2008 ; 366 : 3969 – 87 .
Patrick SM. Reflecting sunlight to reduce climate risk: priorities for research and international cooperation. Council on Foreign Relations . Special Report No. 93, April 2022 (date last accessed 4 December 2022).
Lovins AB. Reinventing Fire . White River Junction, Vermont : Chelsea Green Publishing , 334 p.
Month: | Total Views: |
---|---|
November 2023 | 74,740 |
December 2023 | 14,226 |
January 2024 | 13,452 |
February 2024 | 8,892 |
March 2024 | 8,074 |
April 2024 | 7,567 |
May 2024 | 5,673 |
June 2024 | 4,824 |
July 2024 | 6,021 |
August 2024 | 5,407 |
Email alerts
- Editorial on Hansen et al. ‘ Global warming in the pipeline ’ (this issue)
Citing articles via
- Recommend to Your Librarian
- Advertising & Corporate Services
- Journals Career Network
Affiliations
- Online ISSN 2634-4068
- Copyright © 2024 Oxford University Press
- About Oxford Academic
- Publish journals with us
- University press partners
- What we publish
- New features
- Open access
- Institutional account management
- Rights and permissions
- Get help with access
- Accessibility
- Advertising
- Media enquiries
- Oxford University Press
- Oxford Languages
- University of Oxford
Oxford University Press is a department of the University of Oxford. It furthers the University's objective of excellence in research, scholarship, and education by publishing worldwide
- Copyright © 2024 Oxford University Press
- Cookie settings
- Cookie policy
- Privacy policy
- Legal notice
This Feature Is Available To Subscribers Only
Sign In or Create an Account
This PDF is available to Subscribers Only
For full access to this pdf, sign in to an existing account, or purchase an annual subscription.
Accessibility Links
- Skip to content
- Skip to search IOPscience
- Skip to Journals list
- Accessibility help
- Accessibility Help
Click here to close this panel.

Purpose-led Publishing is a coalition of three not-for-profit publishers in the field of physical sciences: AIP Publishing, the American Physical Society and IOP Publishing.
Together, as publishers that will always put purpose above profit, we have defined a set of industry standards that underpin high-quality, ethical scholarly communications.
We are proudly declaring that science is our only shareholder.
Quantifying the consensus on anthropogenic global warming in the scientific literature
John Cook 1,2,3 , Dana Nuccitelli 2,4 , Sarah A Green 5 , Mark Richardson 6 , Bärbel Winkler 2 , Rob Painting 2 , Robert Way 7 , Peter Jacobs 8 and Andrew Skuce 2,9
Published 15 May 2013 • © 2013 IOP Publishing Ltd Environmental Research Letters , Volume 8 , Number 2 Citation John Cook et al 2013 Environ. Res. Lett. 8 024024 DOI 10.1088/1748-9326/8/2/024024
Article metrics
1462321 Total downloads
Share this article
Author e-mails.
Author affiliations
1 Global Change Institute, University of Queensland, Australia
2 Skeptical Science, Brisbane, Queensland, Australia
3 School of Psychology, University of Western Australia, Australia
4 Tetra Tech, Incorporated, McClellan, CA, USA
5 Department of Chemistry, Michigan Technological University, USA
6 Department of Meteorology, University of Reading, UK
7 Department of Geography, Memorial University of Newfoundland, Canada
8 Department of Environmental Science and Policy, George Mason University, USA
9 Salt Spring Consulting Ltd, Salt Spring Island, BC, Canada
- Received 18 January 2013
- Accepted 22 April 2013
- Published 15 May 2013
Buy this article in print
We analyze the evolution of the scientific consensus on anthropogenic global warming (AGW) in the peer-reviewed scientific literature, examining 11 944 climate abstracts from 1991–2011 matching the topics 'global climate change' or 'global warming'. We find that 66.4% of abstracts expressed no position on AGW, 32.6% endorsed AGW, 0.7% rejected AGW and 0.3% were uncertain about the cause of global warming. Among abstracts expressing a position on AGW, 97.1% endorsed the consensus position that humans are causing global warming. In a second phase of this study, we invited authors to rate their own papers. Compared to abstract ratings, a smaller percentage of self-rated papers expressed no position on AGW (35.5%). Among self-rated papers expressing a position on AGW, 97.2% endorsed the consensus. For both abstract ratings and authors' self-ratings, the percentage of endorsements among papers expressing a position on AGW marginally increased over time. Our analysis indicates that the number of papers rejecting the consensus on AGW is a vanishingly small proportion of the published research.
Export citation and abstract BibTeX RIS
Content from this work may be used under the terms of the Creative Commons Attribution 3.0 licence . Any further distribution of this work must maintain attribution to the author(s) and the title of the work, journal citation and DOI.
1. Introduction
An accurate perception of the degree of scientific consensus is an essential element to public support for climate policy (Ding et al 2011 ). Communicating the scientific consensus also increases people's acceptance that climate change (CC) is happening (Lewandowsky et al 2012 ). Despite numerous indicators of a consensus, there is wide public perception that climate scientists disagree over the fundamental cause of global warming (GW; Leiserowitz et al 2012 , Pew 2012 ). In the most comprehensive analysis performed to date, we have extended the analysis of peer-reviewed climate papers in Oreskes ( 2004 ). We examined a large sample of the scientific literature on global CC, published over a 21 year period, in order to determine the level of scientific consensus that human activity is very likely causing most of the current GW (anthropogenic global warming, or AGW).
Surveys of climate scientists have found strong agreement (97–98%) regarding AGW amongst publishing climate experts (Doran and Zimmerman 2009 , Anderegg et al 2010 ). Repeated surveys of scientists found that scientific agreement about AGW steadily increased from 1996 to 2009 (Bray 2010 ). This is reflected in the increasingly definitive statements issued by the Intergovernmental Panel on Climate Change on the attribution of recent GW (Houghton et al 1996 , 2001 , Solomon et al 2007 ).
The peer-reviewed scientific literature provides a ground-level assessment of the degree of consensus among publishing scientists. An analysis of abstracts published from 1993–2003 matching the search 'global climate change' found that none of 928 papers disagreed with the consensus position on AGW (Oreskes 2004 ). This is consistent with an analysis of citation networks that found a consensus on AGW forming in the early 1990s (Shwed and Bearman 2010 ).
Despite these independent indicators of a scientific consensus, the perception of the US public is that the scientific community still disagrees over the fundamental cause of GW. From 1997 to 2007, public opinion polls have indicated around 60% of the US public believes there is significant disagreement among scientists about whether GW was happening (Nisbet and Myers 2007 ). Similarly, 57% of the US public either disagreed or were unaware that scientists agree that the earth is very likely warming due to human activity (Pew 2012 ).
Through analysis of climate-related papers published from 1991 to 2011, this study provides the most comprehensive analysis of its kind to date in order to quantify and evaluate the level and evolution of consensus over the last two decades.
2. Methodology
This letter was conceived as a 'citizen science' project by volunteers contributing to the Skeptical Science website ( www.skepticalscience.com ). In March 2012, we searched the ISI Web of Science for papers published from 1991–2011 using topic searches for 'global warming' or 'global climate change'. Article type was restricted to 'article', excluding books, discussions, proceedings papers and other document types. The search was updated in May 2012 with papers added to the Web of Science up to that date.
We classified each abstract according to the type of research (category) and degree of endorsement. Written criteria were provided to raters for category (table 1 ) and level of endorsement of AGW (table 2 ). Explicit endorsements were divided into non-quantified (e.g., humans are contributing to global warming without quantifying the contribution) and quantified (e.g., humans are contributing more than 50% of global warming, consistent with the 2007 IPCC statement that most of the global warming since the mid-20th century is very likely due to the observed increase in anthropogenic greenhouse gas concentrations).
Table 1. Definitions of each type of research category.
Category | Description | Example |
---|---|---|
(1) Impacts | Effects and impacts of climate change on the environment, ecosystems or humanity | '...global climate change together with increasing direct impacts of human activities, such as fisheries, are affecting the population dynamics of marine top predators' |
(2) Methods | Focus on measurements and modeling methods, or basic climate science not included in the other categories | 'This paper focuses on automating the task of estimating Polar ice thickness from airborne radar data...' |
(3) Mitigation | Research into lowering CO emissions or atmospheric CO levels | 'This paper presents a new approach for a nationally appropriate mitigation actions framework that can unlock the huge potential for greenhouse gas mitigation in dispersed energy end-use sectors in developing countries' |
(4) Not climate-related | Social science, education, research about people's views on climate | 'This paper discusses the use of multimedia techniques and augmented reality tools to bring across the risks of global climate change' |
(5) Opinion | Not peer-reviewed articles | 'While the world argues about reducing global warming, chemical engineers are getting on with the technology. Charles Butcher has been finding out how to remove carbon dioxide from flue gas' |
(6) Paleoclimate | Examining climate during pre-industrial times | 'Here, we present a pollen-based quantitative temperature reconstruction from the midlatitudes of Australia that spans the last 135 000 years...' |
Table 2. Definitions of each level of endorsement of AGW.
Level of endorsement | Description | Example |
---|---|---|
(1) Explicit endorsement with quantification | Explicitly states that humans are the primary cause of recent global warming | 'The global warming during the 20th century is caused mainly by increasing greenhouse gas concentration especially since the late 1980s' |
(2) Explicit endorsement without quantification | Explicitly states humans are causing global warming or refers to anthropogenic global warming/climate change as a known fact | 'Emissions of a broad range of greenhouse gases of varying lifetimes contribute to global climate change' |
(3) Implicit endorsement | Implies humans are causing global warming. E.g., research assumes greenhouse gas emissions cause warming without explicitly stating humans are the cause | '...carbon sequestration in soil is important for mitigating global climate change' |
(4a) No position | Does not address or mention the cause of global warming | |
(4b) Uncertain | Expresses position that human's role on recent global warming is uncertain/undefined | 'While the extent of human-induced global warming is inconclusive...' |
(5) Implicit rejection | Implies humans have had a minimal impact on global warming without saying so explicitly E.g., proposing a natural mechanism is the main cause of global warming | '...anywhere from a major portion to all of the warming of the 20th century could plausibly result from natural causes according to these results' |
(6) Explicit rejection without quantification | Explicitly minimizes or rejects that humans are causing global warming | '...the global temperature record provides little support for the catastrophic view of the greenhouse effect' |
(7) Explicit rejection with quantification | Explicitly states that humans are causing less than half of global warming | 'The human contribution to the CO content in the atmosphere and the increase in temperature is negligible in comparison with other sources of carbon dioxide emission' |
Abstracts were randomly distributed via a web-based system to raters with only the title and abstract visible. All other information such as author names and affiliations, journal and publishing date were hidden. Each abstract was categorized by two independent, anonymized raters. A team of 12 individuals completed 97.4% (23 061) of the ratings; an additional 12 contributed the remaining 2.6% (607). Initially, 27% of category ratings and 33% of endorsement ratings disagreed. Raters were then allowed to compare and justify or update their rating through the web system, while maintaining anonymity. Following this, 11% of category ratings and 16% of endorsement ratings disagreed; these were then resolved by a third party.
Upon completion of the final ratings, a random sample of 1000 'No Position' category abstracts were re-examined to differentiate those that did not express an opinion from those that take the position that the cause of GW is uncertain. An 'Uncertain' abstract explicitly states that the cause of global warming is not yet determined (e.g., '...the extent of human-induced global warming is inconclusive...') while a 'No Position' abstract makes no statement on AGW.
To complement the abstract analysis, email addresses for 8547 authors were collected, typically from the corresponding author and/or first author. For each year, email addresses were obtained for at least 60% of papers. Authors were emailed an invitation to participate in a survey in which they rated their own published papers (the entire content of the article, not just the abstract) with the same criteria as used by the independent rating team. Details of the survey text are provided in the supplementary information (available at stacks.iop.org/ERL/8/024024/mmedia ).
The ISI search generated 12 465 papers. Eliminating papers that were not peer-reviewed (186), not climate-related (288) or without an abstract (47) reduced the analysis to 11 944 papers written by 29 083 authors and published in 1980 journals. To simplify the analysis, ratings were consolidated into three groups: endorsements (including implicit and explicit; categories 1–3 in table 2 ), no position (category 4) and rejections (including implicit and explicit; categories 5–7).
We examined four metrics to quantify the level of endorsement:
- (1) The percentage of endorsements/rejections/undecideds among all abstracts.
- (2) The percentage of endorsements/rejections/undecideds among only those abstracts expressing a position on AGW.
- (3) The percentage of scientists authoring endorsement/ rejection abstracts among all scientists.
- (4) The same percentage among only those scientists who expressed a position on AGW (table 3 ).
Table 3. Abstract ratings for each level of endorsement, shown as percentage and total number of papers.
Position | % of all abstracts | % among abstracts with AGW position (%) | % of all authors | % among authors with AGW position (%) |
---|---|---|---|---|
Endorse AGW | 32.6% (3896) | 97.1 | 34.8% (10 188) | 98.4 |
No AGW position | 66.4% (7930) | — | 64.6% (18 930) | — |
Reject AGW | 0.7% (78) | 1.9 | 0.4% (124) | 1.2 |
Uncertain on AGW | 0.3% (40) | 1.0 | 0.2% (44) | 0.4 |
3.1. Endorsement percentages from abstract ratings
Among abstracts that expressed a position on AGW, 97.1% endorsed the scientific consensus. Among scientists who expressed a position on AGW in their abstract, 98.4% endorsed the consensus.
The time series of each level of endorsement of the consensus on AGW was analyzed in terms of the number of abstracts (figure 1 (a)) and the percentage of abstracts (figure 1 (b)). Over time, the no position percentage has increased (simple linear regression trend 0.87% ± 0.28% yr −1 , 95% CI, R 2 = 0.66, p < 0.001) and the percentage of papers taking a position on AGW has equally decreased.
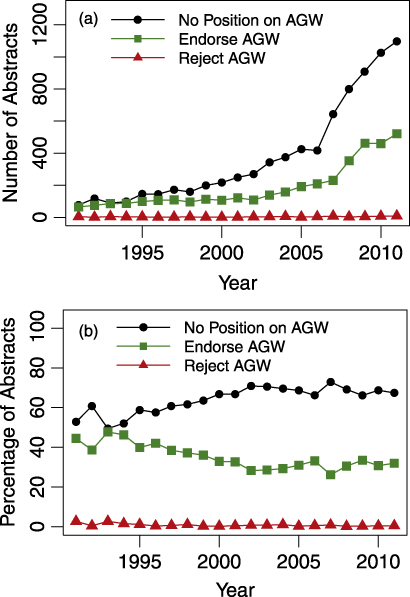
Figure 1. (a) Total number of abstracts categorized into endorsement, rejection and no position. (b) Percentage of endorsement, rejection and no position/undecided abstracts. Uncertain comprise 0.5% of no position abstracts.
Download figure:
The average numbers of authors per endorsement abstract (3.4) and per no position abstract (3.6) are both significantly larger than the average number of authors per rejection abstract (2.0). The scientists originated from 91 countries (identified by email address) with the highest representation from the USA ( N = 2548) followed by the United Kingdom ( N = 546), Germany ( N = 404) and Japan ( N = 379) (see supplementary table S1 for full list, available at stacks.iop.org/ERL/8/024024/mmedia ).
3.2. Endorsement percentages from self-ratings
We emailed 8547 authors an invitation to rate their own papers and received 1200 responses (a 14% response rate). After excluding papers that were not peer-reviewed, not climate-related or had no abstract, 2142 papers received self-ratings from 1189 authors. The self-rated levels of endorsement are shown in table 4 . Among self-rated papers that stated a position on AGW, 97.2% endorsed the consensus. Among self-rated papers not expressing a position on AGW in the abstract, 53.8% were self-rated as endorsing the consensus. Among respondents who authored a paper expressing a view on AGW, 96.4% endorsed the consensus.
Table 4. Self-ratings for each level of endorsement, shown as percentage and total number of papers.
Position | % of all papers | % among papers with AGW position (%) | % of respondents | % among respondents with AGW position (%) |
---|---|---|---|---|
Endorse AGW | 62.7% (1342) | 97.2 | 62.7% (746) | 96.4 |
No AGW position | 35.5% (761) | — | 34.9% (415) | — |
Reject AGW | 1.8% (39) | 2.8 | 2.4% (28) | 3.6 |
a Self-rated papers that endorse AGW have an average endorsement rating less than 4 (1 =explicit endorsement with quantification, 7 = explicit rejection with quantification). b Undecided self-rated papers have an average rating equal to 4. c Rejection self-rated papers have an average rating greater than 4.
Figure 2 (a) shows the level of self-rated endorsement in terms of number of abstracts (the corollary to figure 1 (a)) and figure 2 (b) shows the percentage of abstracts (the corollary to figure 1 (b)). The percentage of self-rated rejection papers decreased (simple linear regression trend −0.25% ± 0.18% yr −1 , 95% CI, R 2 = 0.28, p = 0.01, figure 2 (b)). The time series of self-rated no position and consensus endorsement papers both show no clear trend over time.
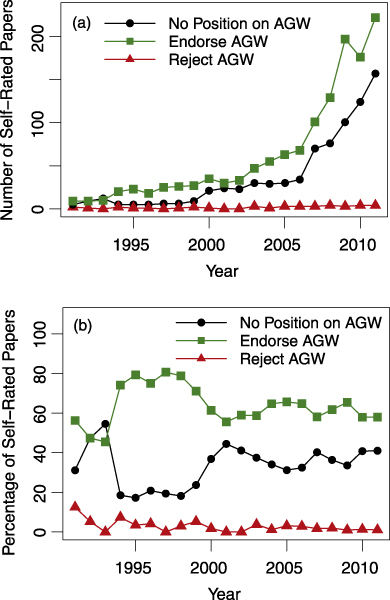
Figure 2. (a) Total number of endorsement, rejection and no position papers as self-rated by authors. Year is the published year of each self-rated paper. (b) Percentage of self-rated endorsement, rejection and no position papers.
A direct comparison of abstract rating versus self-rating endorsement levels for the 2142 papers that received a self-rating is shown in table 5 . More than half of the abstracts that we rated as 'No Position' or 'Undecided' were rated 'Endorse AGW' by the paper's authors.
Table 5. Comparison of our abstract rating to self-rating for papers that received self-ratings.
Position | Abstract rating | Self-rating |
---|---|---|
Endorse AGW | 791 (36.9%) | 1342 (62.7%) |
No AGW position or undecided | 1339 (62.5%) | 761 (35.5%) |
Reject AGW | 12 (0.6%) | 39 (1.8%) |
Figure 3 compares the percentage of papers endorsing the scientific consensus among all papers that express a position endorsing or rejecting the consensus. The year-to-year variability is larger in the self-ratings than in the abstract ratings due to the smaller sample sizes in the early 1990s. The percentage of AGW endorsements for both self-rating and abstract-rated papers increase marginally over time (simple linear regression trends 0.10 ± 0.09% yr −1 , 95% CI, R 2 = 0.20, p = 0.04 for abstracts, 0.35 ± 0.26% yr −1 , 95% CI, R 2 = 0.26, p = 0.02 for self-ratings), with both series approaching approximately 98% endorsements in 2011.
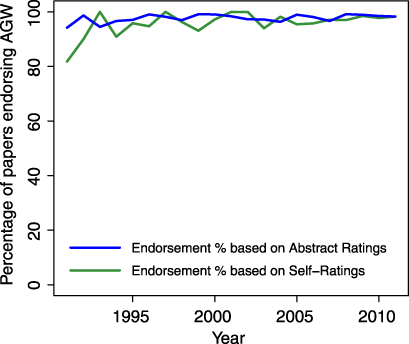
Figure 3. Percentage of papers endorsing the consensus among only papers that express a position endorsing or rejecting the consensus.
4. Discussion
Of note is the large proportion of abstracts that state no position on AGW. This result is expected in consensus situations where scientists ' ...generally focus their discussions on questions that are still disputed or unanswered rather than on matters about which everyone agrees ' (Oreskes 2007 , p 72). This explanation is also consistent with a description of consensus as a 'spiral trajectory' in which ' initially intense contestation generates rapid settlement and induces a spiral of new questions ' (Shwed and Bearman 2010 ); the fundamental science of AGW is no longer controversial among the publishing science community and the remaining debate in the field has moved to other topics. This is supported by the fact that more than half of the self-rated endorsement papers did not express a position on AGW in their abstracts.
The self-ratings by the papers' authors provide insight into the nature of the scientific consensus amongst publishing scientists. For both self-ratings and our abstract ratings, the percentage of endorsements among papers expressing a position on AGW marginally increased over time, consistent with Bray ( 2010 ) in finding a strengthening consensus.
4.1. Sources of uncertainty
The process of determining the level of consensus in the peer-reviewed literature contains several sources of uncertainty, including the representativeness of the sample, lack of clarity in the abstracts and subjectivity in rating the abstracts.
We address the issue of representativeness by selecting the largest sample to date for this type of literature analysis. Nevertheless, 11 944 papers is only a fraction of the climate literature. A Web of Science search for 'climate change' over the same period yields 43 548 papers, while a search for 'climate' yields 128 440 papers. The crowd-sourcing techniques employed in this analysis could be expanded to include more papers. This could facilitate an approach approximating the methods of Doran and Zimmerman ( 2009 ), which measured the level of scientific consensus for varying degrees of expertise in climate science. A similar approach could analyze the level of consensus among climate papers depending on their relevance to the attribution of GW.
Another potential area of uncertainty involved the text of the abstracts themselves. In some cases, ambiguous language made it difficult to ascertain the intended meaning of the authors. Naturally, a short abstract could not be expected to communicate all the details of the full paper. The implementation of the author self-rating process allowed us to look beyond the abstract. A comparison between self-ratings and abstract ratings revealed that categorization based on the abstract alone underestimates the percentage of papers taking a position on AGW.
Lastly, some subjectivity is inherent in the abstract rating process. While criteria for determining ratings were defined prior to the rating period, some clarifications and amendments were required as specific situations presented themselves. Two sources of rating bias can be cited: first, given that the raters themselves endorsed the scientific consensus on AGW, they may have been more likely to classify papers as sharing that endorsement. Second, scientific reticence (Hansen 2007 ) or 'erring on the side of least drama' (ESLD; Brysse et al 2012 ) may have exerted an opposite effect by biasing raters towards a 'no position' classification. These sources of bias were partially addressed by the use of multiple independent raters and by comparing abstract rating results to author self-ratings. A comparison of author ratings of the full papers and abstract ratings reveals a bias toward an under-counting of endorsement papers in the abstract ratings (mean difference 0.6 in units of endorsement level). This mitigated concerns about rater subjectivity, but suggests that scientific reticence and ESLD remain possible biases in the abstract ratings process. The potential impact of initial rating disagreements was also calculated and found to have minimal impact on the level of consensus (see supplemental information, section S1 available at stacks.iop.org/ERL/8/024024/mmedia ).
4.2. Comparisons with previous studies
Our sample encompasses those surveyed by Oreskes ( 2004 ) and Schulte ( 2008 ) and we can therefore directly compare the results. Oreskes ( 2004 ) analyzed 928 papers from 1993 to 2003. Over the same period, we found 932 papers matching the search phrase 'global climate change' (papers continue to be added to the ISI database). From that subset we eliminated 38 papers that were not peer-reviewed, climate-related or had no abstract. Of the remaining 894, none rejected the consensus, consistent with Oreskes' result. Oreskes determined that 75% of papers endorsed the consensus, based on the assumption that mitigation and impact papers implicitly endorse the consensus. By comparison, we found that 28% of the 894 abstracts endorsed AGW while 72% expressed no position. Among the 71 papers that received self-ratings from authors, 69% endorse AGW, comparable to Oreskes' estimate of 75% endorsements.
An analysis of 539 'global climate change' abstracts from the Web of Science database over January 2004 to mid-February 2007 found 45% endorsement and 6% rejection (Schulte 2008 ). Our analysis over a similar period (including all of February 2007) produced 529 papers—the reason for this discrepancy is unclear as Schulte's exact methodology is not provided. Schulte estimated a higher percentage of endorsements and rejections, possibly because the strict methodology we adopted led to a greater number of 'No Position' abstracts. Schulte also found a significantly greater number of rejection papers, including 6 explicit rejections compared to our 0 explicit rejections. See the supplementary information (available at stacks.iop.org/ERL/8/024024/mmedia ) for a tabulated comparison of results. Among 58 self-rated papers, only one (1.7%) rejected AGW in this sample. Over the period of January 2004 to February 2007, among 'global climate change' papers that state a position on AGW, we found 97% endorsements.
5. Conclusion
The public perception of a scientific consensus on AGW is a necessary element in public support for climate policy (Ding et al 2011 ). However, there is a significant gap between public perception and reality, with 57% of the US public either disagreeing or unaware that scientists overwhelmingly agree that the earth is warming due to human activity (Pew 2012 ).
Contributing to this 'consensus gap' are campaigns designed to confuse the public about the level of agreement among climate scientists. In 1991, Western Fuels Association conducted a $510 000 campaign whose primary goal was to ' reposition global warming as theory (not fact) '. A key strategy involved constructing the impression of active scientific debate using dissenting scientists as spokesmen (Oreskes 2010 ). The situation is exacerbated by media treatment of the climate issue, where the normative practice of providing opposing sides with equal attention has allowed a vocal minority to have their views amplified (Boykoff and Boykoff 2004 ). While there are indications that the situation has improved in the UK and USA prestige press (Boykoff 2007 ), the UK tabloid press showed no indication of improvement from 2000 to 2006 (Boykoff and Mansfield 2008 ).
The narrative presented by some dissenters is that the scientific consensus is ' ...on the point of collapse ' (Oddie 2012 ) while ' ...the number of scientific "heretics" is growing with each passing year ' (Allègre et al 2012 ). A systematic, comprehensive review of the literature provides quantitative evidence countering this assertion. The number of papers rejecting AGW is a miniscule proportion of the published research, with the percentage slightly decreasing over time. Among papers expressing a position on AGW, an overwhelming percentage (97.2% based on self-ratings, 97.1% based on abstract ratings) endorses the scientific consensus on AGW.
Acknowledgments
Thanks to James Powell for his invaluable contribution to this analysis, Stephan Lewandowsky for his comments and to those who assisted with collecting email addresses and rating abstracts: Ari Jokimäki, Riccardo Reitano, Rob Honeycutt, Wendy Cook, Phil Scadden, Glenn Tamblyn, Anne-Marie Blackburn, John Hartz, Steve Brown, George Morrison, Alexander C Coulter, Martin B Stolpe (to name just those who are not listed as (co-)author to this paper).
Supplementary data

An official website of the United States government
The .gov means it’s official. Federal government websites often end in .gov or .mil. Before sharing sensitive information, make sure you’re on a federal government site.
The site is secure. The https:// ensures that you are connecting to the official website and that any information you provide is encrypted and transmitted securely.
- Publications
- Account settings
- My Bibliography
- Collections
- Citation manager
Save citation to file
Email citation, add to collections.
- Create a new collection
- Add to an existing collection
Add to My Bibliography
Your saved search, create a file for external citation management software, your rss feed.
- Search in PubMed
- Search in NLM Catalog
- Add to Search
A review of the global climate change impacts, adaptation, and sustainable mitigation measures
Affiliations.
- 1 School of Economics and Management, Nanjing University of Science and Technology, Nanjing, 210094, People's Republic of China.
- 2 Jiangsu Key Laboratory of Chemical Pollution Control and Resources Reuse, School of Environmental and Biological Engineering, Nanjing University of Science and Technology, Xiaolingwei 200, Nanjing, 210094, People's Republic of China.
- 3 School of Economics and Management, Nanjing University of Science and Technology, Nanjing, 210094, People's Republic of China. [email protected].
- 4 School of Business and Economics, North South University, Dhaka, 1229, Bangladesh.
- 5 Department of Journalism, Media and Communications, Daffodil International University, Dhaka, Bangladesh.
- 6 Department of Finance, College of Business Administration, Prince Sattam Bin Abdulaziz University, 173, Alkharj, 11942, Saudi Arabia.
- PMID: 35378646
- PMCID: PMC8978769
- DOI: 10.1007/s11356-022-19718-6
Climate change is a long-lasting change in the weather arrays across tropics to polls. It is a global threat that has embarked on to put stress on various sectors. This study is aimed to conceptually engineer how climate variability is deteriorating the sustainability of diverse sectors worldwide. Specifically, the agricultural sector's vulnerability is a globally concerning scenario, as sufficient production and food supplies are threatened due to irreversible weather fluctuations. In turn, it is challenging the global feeding patterns, particularly in countries with agriculture as an integral part of their economy and total productivity. Climate change has also put the integrity and survival of many species at stake due to shifts in optimum temperature ranges, thereby accelerating biodiversity loss by progressively changing the ecosystem structures. Climate variations increase the likelihood of particular food and waterborne and vector-borne diseases, and a recent example is a coronavirus pandemic. Climate change also accelerates the enigma of antimicrobial resistance, another threat to human health due to the increasing incidence of resistant pathogenic infections. Besides, the global tourism industry is devastated as climate change impacts unfavorable tourism spots. The methodology investigates hypothetical scenarios of climate variability and attempts to describe the quality of evidence to facilitate readers' careful, critical engagement. Secondary data is used to identify sustainability issues such as environmental, social, and economic viability. To better understand the problem, gathered the information in this report from various media outlets, research agencies, policy papers, newspapers, and other sources. This review is a sectorial assessment of climate change mitigation and adaptation approaches worldwide in the aforementioned sectors and the associated economic costs. According to the findings, government involvement is necessary for the country's long-term development through strict accountability of resources and regulations implemented in the past to generate cutting-edge climate policy. Therefore, mitigating the impacts of climate change must be of the utmost importance, and hence, this global threat requires global commitment to address its dreadful implications to ensure global sustenance.
Keywords: Antimicrobial resistance; Biodiversity; COVID-19; Climate change; Mitigation measures.
© 2022. The Author(s), under exclusive licence to Springer-Verlag GmbH Germany, part of Springer Nature.
PubMed Disclaimer
Conflict of interest statement
The authors declare no competing interests.
Methodology search for finalized articles…
Methodology search for finalized articles for investigations. Source : constructed by authors
Framework of the analysis Process.…
Framework of the analysis Process. Source : constructed by authors
Global deaths from natural disasters,…
Global deaths from natural disasters, 1978 to 2020. Source EMDAT (2020)
Schematic description of potential impacts…
Schematic description of potential impacts of climate change on the agriculture sector and…
A typical interaction between the…
A typical interaction between the susceptible and resistant strains. Source: Elsayed et al.…
Sectoral impacts of climate change…
Sectoral impacts of climate change with adaptation and mitigation measures. Source : constructed…
Similar articles
- A comprehensive review of climate change impacts, adaptation, and mitigation on environmental and natural calamities in Pakistan. Hussain M, Butt AR, Uzma F, Ahmed R, Irshad S, Rehman A, Yousaf B. Hussain M, et al. Environ Monit Assess. 2019 Dec 16;192(1):48. doi: 10.1007/s10661-019-7956-4. Environ Monit Assess. 2019. PMID: 31844992 Review.
- Climate Change Adaptation through the Water-Energy-Food Nexus in Southern Africa. Mpandeli S, Naidoo D, Mabhaudhi T, Nhemachena C, Nhamo L, Liphadzi S, Hlahla S, Modi AT. Mpandeli S, et al. Int J Environ Res Public Health. 2018 Oct 19;15(10):2306. doi: 10.3390/ijerph15102306. Int J Environ Res Public Health. 2018. PMID: 30347771 Free PMC article. Review.
- Vector-borne disease and climate change adaptation in African dryland social-ecological systems. Wilcox BA, Echaubard P, de Garine-Wichatitsky M, Ramirez B. Wilcox BA, et al. Infect Dis Poverty. 2019 May 27;8(1):36. doi: 10.1186/s40249-019-0539-3. Infect Dis Poverty. 2019. PMID: 31130141 Free PMC article. Review.
- Health Risks and Costs of Climate Variability and Change. Ebi KL, Hess JJ, Watkiss P. Ebi KL, et al. In: Mock CN, Nugent R, Kobusingye O, Smith KR, editors. Injury Prevention and Environmental Health. 3rd edition. Washington (DC): The International Bank for Reconstruction and Development / The World Bank; 2017 Oct 27. Chapter 8. In: Mock CN, Nugent R, Kobusingye O, Smith KR, editors. Injury Prevention and Environmental Health. 3rd edition. Washington (DC): The International Bank for Reconstruction and Development / The World Bank; 2017 Oct 27. Chapter 8. PMID: 30212118 Free Books & Documents. Review.
- Labour productivity and economic impacts of carbon mitigation: a modelling study and benefit-cost analysis. Zhao M, Huang X, Kjellstrom T, Lee JKW, Otto M, Zhang X, Romanello M, Zhang D, Cai W. Zhao M, et al. Lancet Planet Health. 2022 Dec;6(12):e941-e948. doi: 10.1016/S2542-5196(22)00245-5. Lancet Planet Health. 2022. PMID: 36495888
- Shifts in the gut microbiota of sea urchin Diadema antillarum associated with the 2022 disease outbreak. Ruiz-Barrionuevo JM, Kardas E, Rodríguez-Barreras R, Quiñones-Otero MA, Ruiz-Diaz CP, Toledo-Hernández C, Godoy-Vitorino F. Ruiz-Barrionuevo JM, et al. Front Microbiol. 2024 Jul 29;15:1409729. doi: 10.3389/fmicb.2024.1409729. eCollection 2024. Front Microbiol. 2024. PMID: 39135877 Free PMC article.
- Ni-Based Molecular Sieves Nanomaterials for Dry Methane Reforming: Role of Porous Structure and Active Sites Distribution on Hydrogen Production. Al-Fatesh AS, Ibrahim AA, Fakeeha AH, Osman AI, Alanazi YM, Almubaddel FS, Abasaeed AE. Al-Fatesh AS, et al. Nanomaterials (Basel). 2024 Aug 5;14(15):1320. doi: 10.3390/nano14151320. Nanomaterials (Basel). 2024. PMID: 39120425 Free PMC article.
- Modern technologies and solutions to enhance surveillance and response systems for emerging zoonotic diseases. Zhang L, Guo W, Lv C. Zhang L, et al. Sci One Health. 2023 Dec 12;3:100061. doi: 10.1016/j.soh.2023.100061. eCollection 2024. Sci One Health. 2023. PMID: 39077381 Free PMC article. Review.
- Decarbonizing progress: Exploring the nexus of renewable energy, digital economy, and economic development in South American countries. Li W, Nadeem M. Li W, et al. Heliyon. 2024 Jun 25;10(13):e33446. doi: 10.1016/j.heliyon.2024.e33446. eCollection 2024 Jul 15. Heliyon. 2024. PMID: 39071722 Free PMC article.
- Shelf Life of Minced Pork in Vacuum-Adsorbed Carvacrol@Natural Zeolite Nanohybrids and Poly-Lactic Acid/Triethyl Citrate/Carvacrol@Natural Zeolite Self-Healable Active Packaging Films. Karabagias VK, Giannakas AE, Andritsos ND, Leontiou AA, Moschovas D, Karydis-Messinis A, Avgeropoulos A, Zafeiropoulos NE, Proestos C, Salmas CE. Karabagias VK, et al. Antioxidants (Basel). 2024 Jun 27;13(7):776. doi: 10.3390/antiox13070776. Antioxidants (Basel). 2024. PMID: 39061844 Free PMC article.
- Abbass K, Begum H, Alam ASA, Awang AH, Abdelsalam MK, Egdair IMM, Wahid R (2022) Fresh Insight through a Keynesian Theory Approach to Investigate the Economic Impact of the COVID-19 Pandemic in Pakistan. Sustain 14(3):1054
- Abbass K, Niazi AAK, Qazi TF, Basit A, Song H (2021a) The aftermath of COVID-19 pandemic period: barriers in implementation of social distancing at workplace. Library Hi Tech
- Abbass K, Song H, Khan F, Begum H, Asif M (2021b) Fresh insight through the VAR approach to investigate the effects of fiscal policy on environmental pollution in Pakistan. Environ Scie Poll Res 1–14 - PubMed
- Abbass K, Song H, Shah SM, Aziz B. Determinants of Stock Return for Non-Financial Sector: Evidence from Energy Sector of Pakistan. J Bus Fin Aff. 2019;8(370):2167–0234.
- Abbass K, Tanveer A, Huaming S, Khatiya AA (2021c) Impact of financial resources utilization on firm performance: a case of SMEs working in Pakistan
Publication types
- Search in MeSH
Related information
Linkout - more resources, full text sources.
- Europe PubMed Central
- PubMed Central
- MedlinePlus Health Information
Miscellaneous
- NCI CPTAC Assay Portal
- Citation Manager
NCBI Literature Resources
MeSH PMC Bookshelf Disclaimer
The PubMed wordmark and PubMed logo are registered trademarks of the U.S. Department of Health and Human Services (HHS). Unauthorized use of these marks is strictly prohibited.
IEEE Account
- Change Username/Password
- Update Address
Purchase Details
- Payment Options
- Order History
- View Purchased Documents
Profile Information
- Communications Preferences
- Profession and Education
- Technical Interests
- US & Canada: +1 800 678 4333
- Worldwide: +1 732 981 0060
- Contact & Support
- About IEEE Xplore
- Accessibility
- Terms of Use
- Nondiscrimination Policy
- Privacy & Opting Out of Cookies
A not-for-profit organization, IEEE is the world's largest technical professional organization dedicated to advancing technology for the benefit of humanity. © Copyright 2024 IEEE - All rights reserved. Use of this web site signifies your agreement to the terms and conditions.
- Open access
- Published: 15 October 2020
Climate change: Does international research fulfill global demands and necessities?
- Doris Klingelhöfer ORCID: orcid.org/0000-0003-0716-9872 1 ,
- Ruth Müller 1 , 2 ,
- Markus Braun 1 ,
- Dörthe Brüggmann 1 &
- David A. Groneberg 1
Environmental Sciences Europe volume 32 , Article number: 137 ( 2020 ) Cite this article
49k Accesses
32 Citations
34 Altmetric
Metrics details
Climate change is safe to be one of the biggest challenges of mankind. Human activities, especially the combustion of fossil fuels, contribute to the increase of greenhouse gases in the atmosphere and thus to the pace of climate change. The effects of climate change are already being felt, and the resulting damage will most likely be enormous worldwide. Because global impacts vary widely and will lead to very different national vulnerability to climate impacts, each country, depending on its economic background, has different options to ward off negative impacts. Decisions have to be made to mitigate climate consequences according to the preparedness and the vulnerability of countries against the presumed impacts. This requires a profound scientific basis. To provide sound background information, a bibliometric study was conducted to present global research on climate change using established and specific parameters. Bibliometric standard parameters, established socioeconomic values, and climate change specific indices were used for the analyses. This allowed us to provide an overall picture of the global research pattern not only in terms of general aspects, but also in terms of climate change impacts, its effects and regional differences. For this purpose, we choose representative indices, such as the CO 2 emissions for the responsibility of countries, the global climate risk index as a combination value for the different types of damage that countries can expect, the increase in sea level as a specific parameter as a measure of the huge global environmental impacts, and the readiness and vulnerability index for the different circumstances of individual countries under which climate change will take place. We hope to have thus made a comprehensive and representative selection of specific parameters that is sufficient to map the global research landscape. We have supplemented the methodology accordingly.
In terms of absolute publication numbers, the USA was the leading country, followed by the UK, and China in 3rd place. The steep rise in Chinese publication numbers over time came into view, while their citation numbers are relatively low. Scandinavian countries were leading regarding their publication numbers related to CO 2 emission and socioeconomic indices. Only three developing countries stand out in all analyses: Costa Rica, the Fiji Atoll, and Zimbabwe, although it is here that the climate impact will be greatest. A positive correlation between countries’ preparedness for the impacts of climate change and their publication numbers could be shown, while the correlation between countries’ vulnerability and their publication numbers was negative.
Conclusions
We could show that there exists an inequity between national research efforts according to the publication output and the demands and necessities of countries related to their socioeconomic status. This inequity calls for a rethink, a different approach, and a different policy to improve countries' preparedness and mitigation capacity, which requires the inclusion of the most affected regions of the world in a strengthened international cooperation network.
Particularly in the western world, public awareness of the consequences of climate change has reached a high level. Before the appearance of the coronavirus pandemics (SARS-CoV 2), hardly any news broadcast in the western world could do without commentary on climate change. Every week millions of pupils and students around the world demonstrated all for a strict ecological regimen of all governments to ensure the 2 °C target of the Paris Agreement [ 42 ]. In “Corona times” the effects of climate change seem almost forgotten by the public, although many scientists have already explained the connection between climate change and the increase in zoonoses [ 24 , 36 ]. Besides, the negative effects of climate change will certainly be more permanent and severe than the temporary damage of a pandemic; however, severe it may be.
Climate change will undoubtedly affect the entire planet and calls for international collective action. Shifts in wind patterns, the average temperature, or the amount of precipitation and frequency of extreme weather events will endanger the health, the food, and the water supply for humans. Those risks are directly linked to the reduction in biological diversity and the extinction of species that challenge most parts of the world. The impacts of climate change will lead to socioeconomic and political instability, which will change the living conditions of many communities.
The global climate has always been changing. However, the enormous problems are caused by the speed with which changes due to human intervention are progressing, and greenhouse gas concentrations have reached levels never before experienced by mankind. Although climate change has officially been considered the most hazardous global risk so far, the recent Conference of Parties (COP) in Madrid failed to achieve binding measures for nations.
But time is running. Solutions must be found to mitigate the consequences of climate change. Governments must react and be prepared for the worst future scenarios that require strategies without national borders. Climate change affects every country in different ways, and the ways in which countries can prepare for it or mitigate its impacts vary widely.
But what has actually happened so far? Anthropogenic activities, in particular the combustion of fossil fuels, have accelerated the rise in carbon dioxide emissions and thus the increase in global warming, with tangible impacts on humans, animals, and the ecological balance around the world [ 45 ]. The immediate environmental consequence of global warming is the increase in natural disasters, e.g., melting glaciers, more extreme and more frequent floods, wildfires, storms, and droughts or heatwaves. The indirect consequences include threats to human health, and the reduction of biodiversity and habitable areas, leading to migration and deterioration of community, public health, and socioeconomic conditions in most countries of the world [ 45 ].
Reliable estimation of the extent of these impacts is at the heart of research and forms the basis for all mitigation strategies at governmental, economic, scientific, or personal levels.
A sound research database is necessary for sustainable approaches for assessing and mitigating climate change impacts. The research on the climate change focuses on a wide range of areas and modeling approaches to consider different future carbon dioxide (CO 2 ) emission scenarios to assess local and regional global warming. CO 2 is a major component of the global carbon cycle and both a natural part of the atmosphere and an essential greenhouse gas. It is mainly through the combustion of fossil fuel that humans influence the amount of CO 2 emission and thus contributes to global warming.
For this, experts who cover all areas of climate change are in demand. These areas range from ecology, life sciences, meteorology, health care, social, and economic sciences, mathematics and computer science to energy, food, and transport. Interdisciplinary approaches deliver huge amounts of data to create reliable future scenarios. They should provide a comprehensive understanding of the problem and possible measures at all levels. All models show significant geographical differences and illustrate the enormous burden on many developing countries. However, there is no in-depth analysis evaluating global research efforts on climate change including climate change-specific parameters, that provides a comprehensive picture with specific geographical and chronological patterns of scientific publications and the resulting needs and requirements for scientific action. Therefore, the present study focuses on the evaluation of the global and national publication output on climatic change to depict structures and international developments using bibliometric analyses. Metadata analysis allows a comprehensive assessment of the global scientific landscape because all countries are vulnerable to the impacts of climate change to varying degrees because of their natural and socioeconomic conditions.
Building on other bibliometric studies [ 2 , 35 ], which also show the publication output of countries in the field of climate change, this analysis interprets global scientific output using country-specific indices relevant to climate change to present the world map accordingly [ 9 , 13 , 33 ]. The resulting implications help to answer the question of whether international research and networking on climate change meet global requirements and necessities given the current and predicted impacts on all regions and all areas of life. Thus, the interpretation of the results can enable decision makers, funders, scientists, and other stakeholders to develop concepts for future research based on carefully evaluated metadata.
Methodological platform
A representative and qualitative database has been built up, providing comprehensive metadata on the past and present scientific landscape of climate change research, its incentives, its benchmarks, and its challenges and requirements. The applied method is integrated into the bibliometric platform New Quality and Quantity Indices in Science (NewQIS), which was initiated in 2009 to provide in-depth data of the publication output on a variety of life science and biomedical topics [ 14 , 17 ]. The approach combines the application of publication and contextual factors with state-of-the-art visualization techniques. The Core Collection Indices of Web of Science (WoS), which represent one of the most important scientific literature databases, are used as data sources. In addition, WoS provides citation parameters for advanced data interpretation and quality assessment via the Journal Citation Report (JCR) and the Journal Impact Factor (JIF).
Search strategy, data acquisition, and correction
The quality of the database depends on the appropriateness of the search strategy applied. The search term must involve all important synonyms. For this study the terms: “climat* change”, “global warming”, and “greenhouse effect” were applied. The asterisk acts as a wild card and was used to search for terms with different endings. To retrieve only the original research publications, only data from the publication type “Articles” was downloaded. The Art and Humanity entries were excluded. No limitation of the evaluation period was made so that all articles from 1900 to 2020 were included in the analysis (Fig. 1 ).
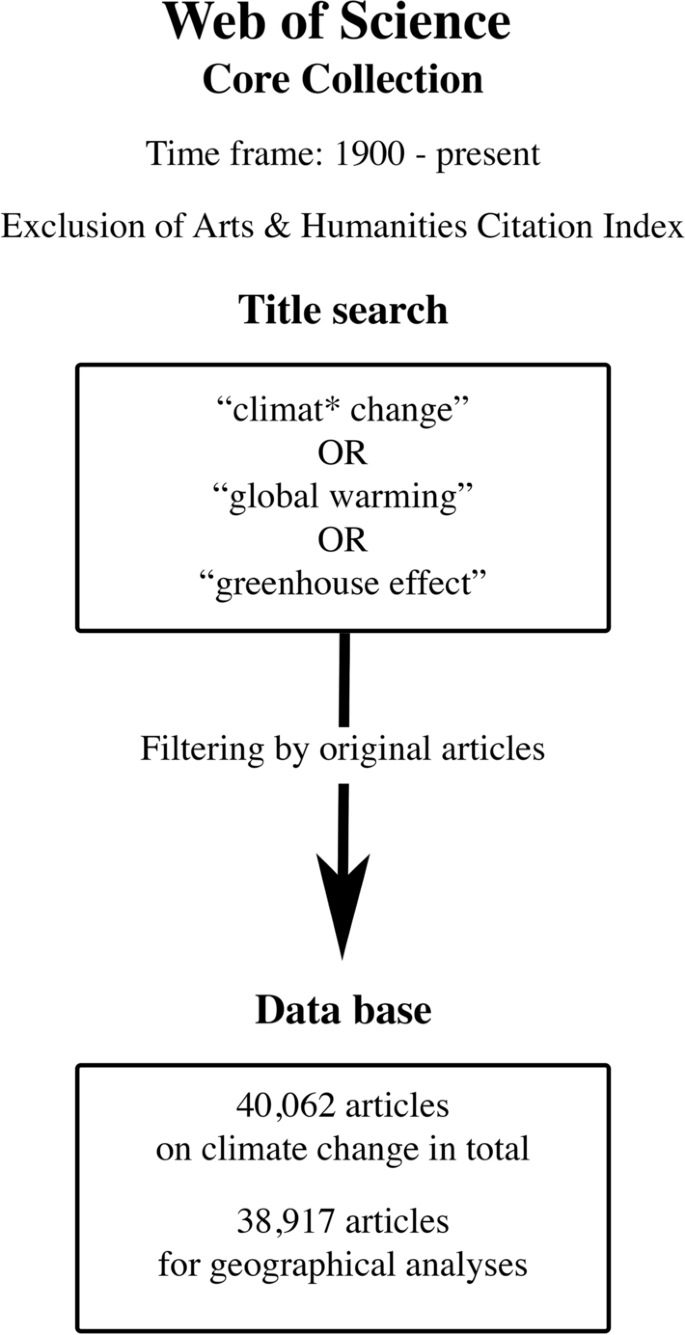
Procedure for generating the analysis database
The aim was to decimate thematically incorrect entries and maximize correct ones. The risk of an unrepresentative database has been reduced by searching in the title of the manuscripts, even if e-data resulting from the search strategy cannot include all indexed articles. The metadata, sorted by various keyed information, was downloaded and saved as an MS-Access database. To unify different designations of data, e.g., the names of authors and their institutional affiliation, a standardization had to be carried out with the help of a specially developed application. For the standardization of institutions, a quantity of at least 200 articles in a regional context must be achieved. A threshold of at least 20 articles on climate change was set for authors. By applying those thresholds, it was possible to completely adjust all entries for institutions and authors above this value. Also, the names of the assigned subject areas had to be adapted and standardized due to missing spaces or typing errors. In doing so, all entries could be corrected without using a threshold value.
Analysis parameters
The resulting database consists of a large number of bibliometric parameters. The research topics were clustered based on the keywords that occur at least 650 times (threshold) using the application VOSviewer [ 44 ].
Chronological analyses were carried out to evaluate the development of research (number of articles), research incentives (number of citations). In addition, geographical analyses were conducted to identify the main actors (countries with the most cited publications, most publishing institutions), and their international networking. The average citation rate of the countries is calculated by dividing the number of citations received by the number of the publication on climate change.
However, the evaluation of the absolute numbers does not allow an assessment of the development of publication shares and the current distribution of countries’ research output on climate change issues. Therefore, the evaluation period of the last 30 years was divided into 5-year intervals for further analysis, and the ten most publishing countries were analyzed.
By linking socioeconomic characteristics and citation parameters, important additional statements on country-specific publication activities on climate change can be made.
The country-specific number of articles was put in relation to (1) Demography: total population in million inhabitants ( R POP ) [ 39 ], (2) Socioeconomic status: gross domestic product (GDP) in billion US-Dollars ( R GDP ) [ 38 ], and (3) Research investment parameters: number of researchers in FTE (full-time equivalents) [ 40 ], expenditures on research and development (R&D) (personnel in FTE) [ 40 ], and gross expenditures for R&D (GERD) in PPP$ (purchasing power parity in US-Dollars) [ 40 ]. For all ratios, a minimum threshold of at least 30 articles was applied to avoid distortions due to extreme values.
For a more specific assessment of the national research contribution, it seems appropriate to include relevant country-specific indicators related to climate change. For this purpose, we select representative indices to put them in relation to the research output of the countries. CO 2 emissions represent the responsibility of countries, the Global Climate Risk Index acts as a composite value for the different types of damage that countries can expect, the rise in sea level as a measure of the enormous global environmental impact, and the readiness and vulnerability index for the different circumstances of the individual countries under which climate change will occur.
Carbon dioxide (CO 2 ) emission in tons per year [ 33 ]: The integration of the CO 2 emissions of the countries was done by calculating the relation of the number of articles to CO 2 emissions in billions of tons (threshold = 300).
The Global Climate Risk Index (CRI): The CRI was launched by German Watch and published in its 15th edition 2020. It assesses the extent to which countries have been suffering from weather incidences [ 9 ]. The CRI provides data for the last 20 years as an average value and also for individual years. The existing data on the weather vulnerability, measured in fatalities per country, and losses in US dollars could indicate the expected increase in extreme events due to climate change and help to mitigate the impacts.
Sea-level rise: For the analysis of the number of people living on vulnerable land due to prognosticated sea level rise [ 19 ], we have taken the values of the average number of fatalities per 100,000 inhabitants from 1999 to 2018 as reference quantity. To estimate the resulting sea-level rise, the US National Aeronautics and Space Administration (NASA) created the digital elevation model (DEM) SRTM ( Shuttle Radar Topography Mission ). The here utilized CoastalDEM is a development based on the neural networks to reduce SRTM errors resulting from its limitation with respect to terrain elevations (important for densely populated areas) by regression analysis [ 19 ]. There are several prospective scenarios, based on the 5th IPCC report [ 15 ], which are based on the Representative Concentration Pathways (RCP) models 2.6, 4.5, and 8.5 leading to different degrees of global temperature rise. These scenarios presuppose different greenhouse gas concentrations. RCP 8.5 would lead to a rise of 4 °C, while RCP 4.5 would lead to a rise of 2.6 °C, and the target limit of 2 °C set by the Paris Agreement can be realized by the RCP 2.6 scenario—always as compared to pre-industrial times [ 10 ]. In addition, the Sea Level Rise Modell K17 is a nonprobabilistic projection that incorporates physical models of ice sheet dynamics [ 18 ]. Furthermore, the applied model data refer to the forecast for the year 2100 and include the local 1-year coastal flood return level [ 19 ]. For our analysis, we chose the K17 model, CoastalDEM, RCP 4.5 for the year 2100.
Readiness and vulnerability index: To assess the differences between the individual countries, the Notre Dame Global Adaptation Initiative (ND-GAIN) developed a country index that provides data on countries’ vulnerability to climate disruption and their readiness to improve resilience by “leverage of private and public sector investments”. The index combines 74 variables to define the ranking for 192 countries [ 27 ].
Visualization of results
The results of the keyword cluster analysis were presented using the VOSviewer software developed by van Eck and Waltman [ 44 ]. The occurrence of keywords was visualized by a network of nodes and connecting lines representing the different colored clusters and their combinations.
The geographical findings of this study were partially visualized by the creation of anamorphic cartograms using Gastner and Newman’s method of density equalizing map projections (DEMP) [ 12 ]. Methodically, these DEMPs reduce or enlarge the country sizes according to the value of the evaluation parameter, following the physical principle of density compensation by diffusion balance in each country. To maintain the basic structure of the world map, mean values are calculated and assigned to oceans and Antarctica. With an ArcGIS tool (mapping software for geographic information systems), which is based on the algorithms of the DEMP method, geographic data can be visualized by generating distorted maps. The DEMPs generated in this way allow a quick visual acquisition of the extensive data and concentration on the essential.
Methodological limitations and strengths
Although being a sophisticated and widely applied method, some limiting points need to be recognized and discussed.
The quality and representativeness of the retrieved metadata depend on the one hand on the technical and bibliographic conditions of the source database and on the other hand on the care taken in generating the search strategy. In this case, WoS was used as a data source. It should be noted that WoS is English biased, as most of the indexed journals are English-language journals. Furthermore, the citation number given is prone to various errors, e.g., incorrect citation behavior or self-citation, so that its significance for the quality of research needs to be discussed. Although the strategy of searching only in the title of the publications resulted in a reduced data quantity, this is justified by the higher representativeness of the data sets. The additional search in the abstracts and keywords would lead to the inclusion of a large number of false entries that would not provide valid figures. Therefore, choosing a title search strategy allows the creation of a valid, albeit not all-encompassing, database.
Some data records had to be corrected manually, e.g., institutions and subject areas. Although the unification of the subject areas in the overall database could be carried out exactly, the merging of different labeled affiliations belonging together was not 100% possible. Therefore, a threshold has been applied in a geographical approach, so that only those geographical entity, e.g., cities, with at least 200 articles on climate change were subject to in-depth corrections. However, the exact number of publishing institutions could not be determined.
The visualization of the results utilizing DEMPs is limited by the physical principles of the technique so that some small island countries could not be represented in the respective figures.
All the results are based on the evaluation database, which consists of a total of 40,062 articles on climate change identified and extracted from WoS.
Research focal points
In total, 45 keywords from three main clusters could be identified (Fig. 2 a). First, articles relating to environmental and ecological issues can be grouped together, with "impacts" being the most commonly used term in the cluster. Secondly, all articles dealing with modeling and simulation can be grouped. In this second keyword cluster, the terms “temperature”, “model”, and “variability” appeared most frequently. Thirdly, all articles on social, political, and management issues can be grouped in one cluster. The umbrella term “climate change” was assigned to this group and is the most frequently used keyword in the analysis. In addition, the terms “adaption” and “vulnerability” have been used most frequently in the third keyword cluster.
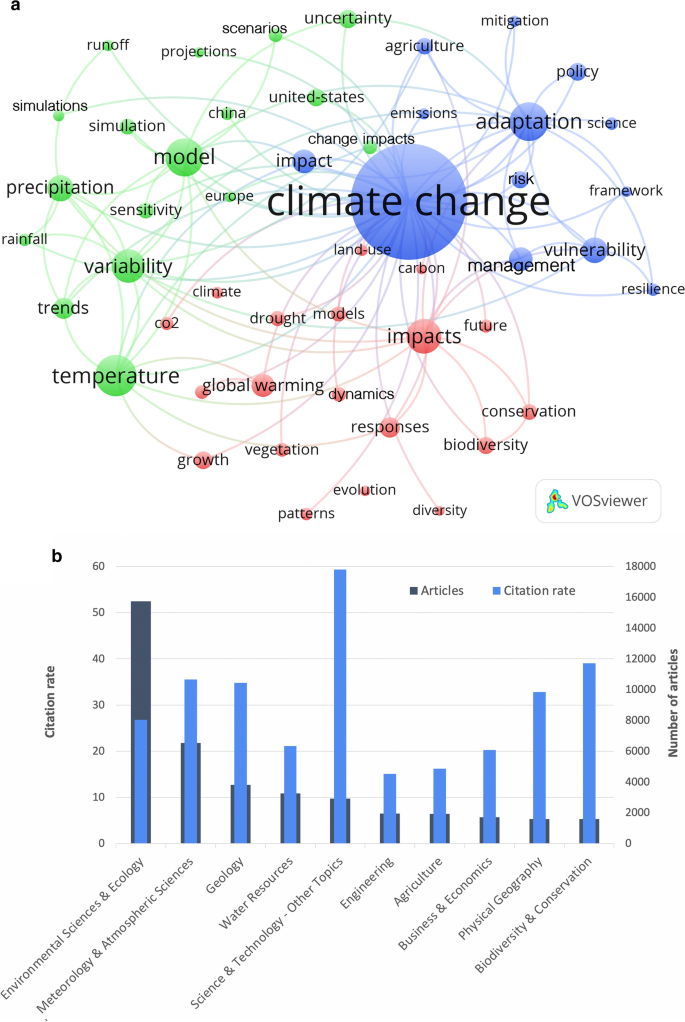
Research foci. a Clusters of author’s keywords with at least 650 occurrences. Red: environmental and ecological issues, green: modeling and simulation issues, blue: social and management issues. b Most assigned subject areas according to Web of Science categories with number of articles and average citation rate (number of citations / number of articles)
The main subject areas (WoS research areas) are shown in figure Fig. 2 b with the numbers of articles ( n ) assigned to them and their average citation rates. By far the most assigned subject area was Environmental Science and Ecology ( n = 15,741). Meteorology and Atmospheric Science ( n = 6522) followed with less than half of assigned articles. Ranks 3 to 5 were occupied by Geology ( n = 3806), Water Resources ( n = 3247), and Science and Technology—Other Topics ( n = 2916). Apart from ecological issues, the most frequently assigned subject areas ( Business and Economics: n = 1710 , Government and Law: n = 1493 , Public Administration: n = 936 ) focus on economics and political issues, which represent the blue cluster in Fig. 1 a. In principle, the articles are distributed over the three main subject areas clusters that distinguish between scenario modeling, risk analysis, and mitigation, respectively, adaption measurements. From these results, the main foci of climate change research can be identified. In summary, articles on modeling and simulation of scenarios for consequences of climate change under different conditions have been developed resulting in ecological and socioeconomic impacts, which in turn form the basis for mitigation and adaption measures on climate change.
It is noteworthy concerning the average citation rate (cr) of the research areas that the highest rates reached the areas Science and Technology—Other Topics (cr = 59.37), Biodiversity and Conservation (cr = 39.01), Geography (cr = 38.85), and Meteorology and Atmospheric Science (cr = 35.54), while the most assigned area Environmental Science and Ecology achieved an average of only cr = 26.80. Among the ten most frequently assigned subject areas, Public Administration (cr = 13.49) and Government and Law ranked last (cr = 10.69).
Evolution of publication output over time
The vast majority of articles on climate change (92.17%) has been published since the year 2000 ( n = 36,925) (Fig. 3 ). However, the first publication that meets the search criteria was published as early as 1910. Annual publication numbers remained in single digits until the mid-1970s. Only at the end of the 1980s, the numbers reach yearly amounts above n = 100. A steep increase in research activity can be observed from 2003 onwards when the trend followed an exponential course, which reached a small peak in 2011 and is still rising exponentially until today (Fig. 3 a). This development can be illustrated even more clearly by looking at the numbers in relation to the absolute number of articles indexed in the Science Citation Index (SCI) (Fig. 2 b). The gradual increase in research interest is also reflected in the steep relative increases in these years, calculated with the annual number of articles on climate change per 10,000 articles listed in the Science Citation Index (SCI) (Fig. 3 b). Until 1988 and between 1992 and 2003, the upward trend of climate change research is similar to that for all articles indexed in the SCI.
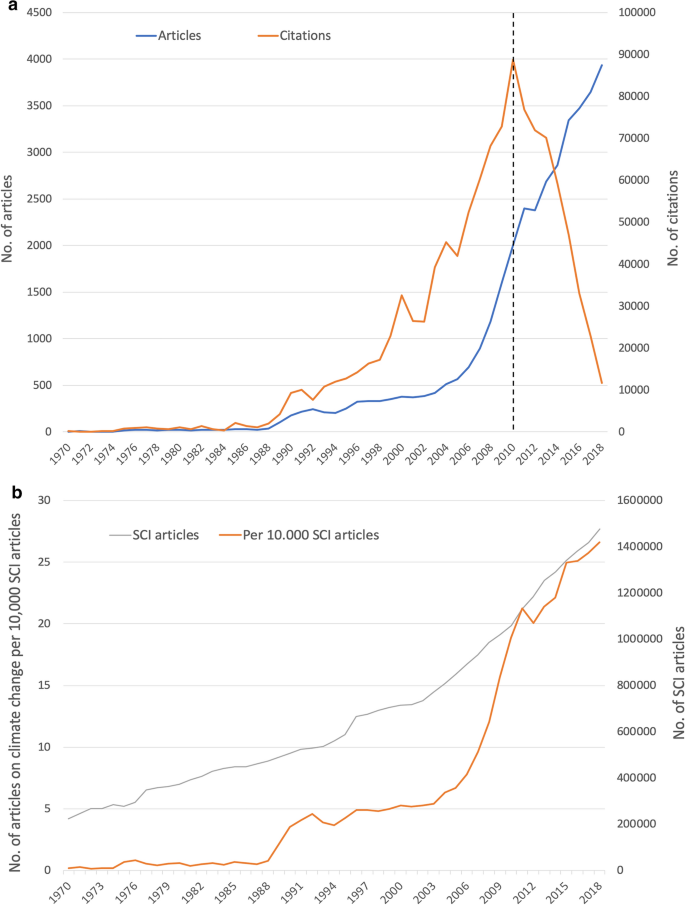
Chronological development of articles on climate change from 1970 to 2018. a Number of articles on climate change and their citations. Dashed line: Cited Half-Life. b Number of all indexed SCI articles (Science Citation Index of Web of Science) and number of articles on climate change per 10,000 SCI articles
Analog to the development of the number of articles, the number of citations (c) also increased significantly since 1988, with peaks in the years 1991 ( c = 10,106), 2000 ( c = 32,612), 2004 ( c = 45,177), and the preliminary maximum of c = 88,747 in 2010. Afterward, the citation numbers dropped again significantly. This is because little time has elapsed since the articles were published to generate citations. This effect is known as Cited Half-Life (CHL) and refers to a period of about eight years for the life sciences, which is needed for the articles to reach half of the total number of citations (Fig. 3 a) [ 21 ].
Among the ten most frequently cited articles in the database, 80% stem can from the USA ( n = 8) and 20% from the UK ( n = 2). All of those ten articles were published after 2000, and mainly in the renowned journals Nature ( n = 5) and Science ( n = 2) (Tables 1 , 2 ). The publication years 2000, 2003, and 2010 can be logically associated with the research increase shown in Fig. 2 a.
Leading institutions
The 15 most publishing institutions on climate change are located exclusively in the northern hemisphere (Table 2 ). Almost half of the most publishing institutions are US-American (7 institutions), 3 others are British, 2 are Dutch, and 1 institution is located in China, Switzerland, and Germany respectively. The Chinese Academy of Science (CAS) was the most publishing institution on climate change with n = 1333 articles, followed by the University of London ( n = 680), which published only half the amount. The US Department of Agriculture (USDA) followed with n = 588 articles. In 4th place was the British University of Oxford ( n = 452), followed by the Dutch Wageningen University ( n = 442) and the US University of Washington ( n = 426). When looking at the average citation rate of the most publishing institutions, the order is different. With the highest value of almost 100, the US National Center for Atmospheric Research (cr = 98.94) led the ranking, followed by the British University of East Anglia (cr = 89.16), and the US Columbia University (cr = 75.16). The articles of the CAS ranked last among the leading 15 institutions (cr = 21.29).
Global landscape of publication output
Not all articles out of the entire database could be assigned to a country of origin due to missing metadata before 1973. Coming from 186 countries or autonomous regions, n = 38,917 articles could thus be included in the database and analyzed in terms of geographical parameters.
The most publishing country was the USA with n = 12,637 articles on climate change, followed by the United Kingdom (UK) with less than half as many articles ( n = 5524). China was placed 3 rd with n = 3508, followed by Australia ( n = 3349), Germany ( n = 3238, and Canada ( n = 3126) (Fig. 4 a).
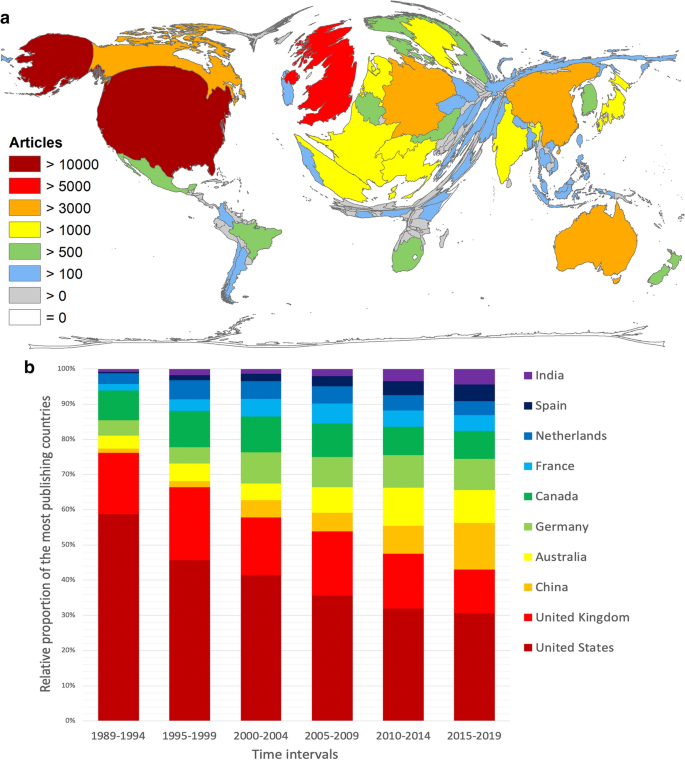
The most publishing countries. a Density equalizing map projection of the number of articles. b Relative share of the most publishing countries in 5-year intervals from 1998 to 2019
Looking at the share of the most publishing countries in 5-year intervals (Fig. 4 b), the USA conducted more than 50% of the research on climate change in the first evaluation interval from 1989 to 1994. In the last interval from 2015 to 2019, the share of US articles decreased to 30%, whereas the absolute numbers increased almost tenfold. The relative share of the UK fell also from 20.64% to 12.42% between 1995 and 2019, during which time it lost its second rank to China that contributed an increasing share from 1.23% to 13.27% throughout the whole evaluation period. The share of Australian, German, Spanish, and Indian articles also increased slightly over time, while the shares of Canadian, French, and Netherlandic articles remained more or less the same.
The distribution of the number of citations follows a similar pattern with the exception of China, which here falls to rank 8 ( c = 66,844). The USA received by far the most citations ( c = 513,888), followed by the UK ( c = 243,261), Australia ( c = 108,054), Canada (c = 107,713), and Germany ( c = 107,335) (Fig. 5 a).
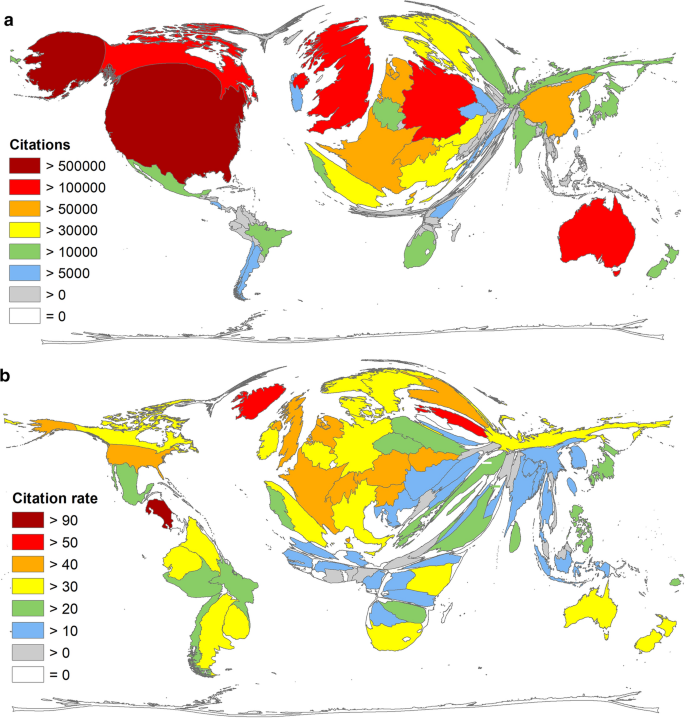
Citation-specific parameters for articles on climate change. a Number of citations per country. b Articles/Citation rate of articles on climate change per country (threshold 30 articles)
When evaluating the average citation rate (cr) per country with more than 30 articles on climate change (threshold), Costa Rica was in first place (cr = 93.89, n = 67), followed by Estonia (cr = 55, n = 66), Iceland (cr = 50.15, n = 47), Austria (cr = 46.77, n = 668), and Switzerland (cr = 45.94, n = 1126). The UK ranked 16th (cr = 44.04), the USA 21st (cr = 40.66), Canada 35th (cr = 34.46), Germany 40th (cr = 33.15), and Australia 41st (cr = 32.26) (Fig. 5 b).
Inclusion of socioeconomic parameters
The analysis of socioeconomic parameters of the publishing countries on climate change showed a divergent ranking.
In terms of the inclusion of the countries’ population size [ 39 ] (number of articles/population in million inhabitants = R POP ) the following order emerged: Norway ( R POP = 174.16), Australia ( R POP = 145.65), Denmark ( R POP = 142.12), Iceland ( R POP = 139.93), Switzerland ( R POP = 137.66). The most publishing countries were ranked lower: the USA ranked 20th ( R POP = 39.00), the UK ranked 13th ( R POP = 85.73), China ranked 67th ( R POP = 2.55), and Germany ranked 18th ( R POP = 40.11) (Fig. 6 a).
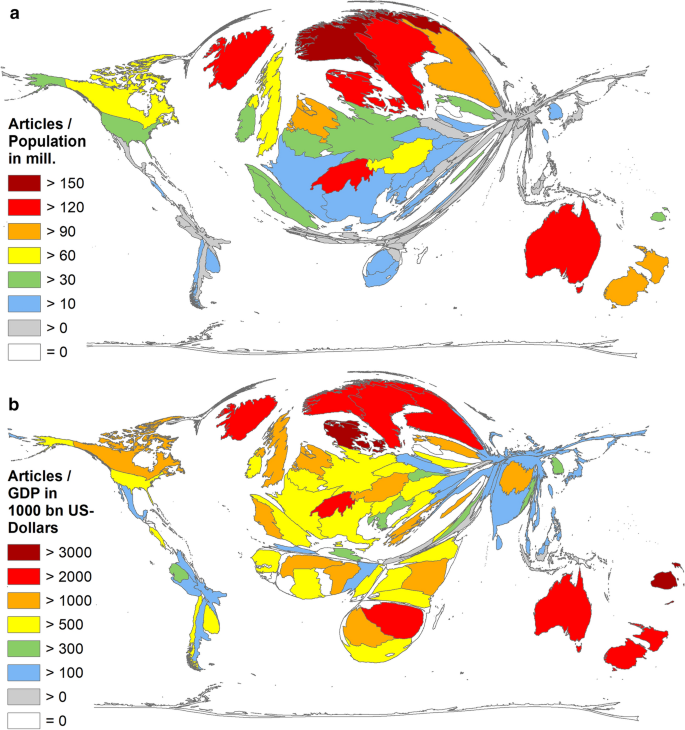
Ratio of socio-economic parameters (threshold 30 articles). a Country-specific ratios of the number of articles on climate change and the countries’ population size in million inhabitants [39]. B) Country-specific ratios of the number of articles on climate change and the Gross Domestic Product (GDP) in 1000 billion US-Dollars [38]
In terms of the economic status, the South Pacific island state Fiji led the range of countries with more than 30 articles on climate change (threshold) with a ratio of the numbers of articles and the GDP in billion US-Dollars [ 38 ] ( R GDP ) with R GDP = 6329.11, followed by Denmark ( R GDP = 3002.26), New Zealand ( R GDP = 2991.99), Iceland ( R GDP = 2910.22), and Australia ( R GDP = 2816.65) (Fig. 6 b). It was also surprising that the African country Zimbabwe was placed among the top ten countries and reached 7th place ( R GDP = 2541.48). In terms of socioeconomic analysis, other developing countries such as Nepal and some African countries (Kenya, Benin) achieved also ranks among the leading 20 countries.
Besides Australia, the UK reached the second highest ratio of the most publishing countries and ranked 11th, Canada ranked 13th, Germany 30th. The USA was only in 37th position.
The inclusion of science-related parameters [ 40 ]. listed New Zealand first (Table 3 ) with R GERD (number of articles/gross expenditure for research and development in current PPP (purchasing power parity) US dollars) = 244.34. Australia ranked 2nd ( R GERD = 157.98), followed by Norway ( R GERD = 133.77), South Africa ( R GERD = 120.36), and UK ( R GERD = 115.54). Germany only achieved rank 22nd ( R GERD = 25.47), and the USA ranked 23rd ( R GERD = 23.26).
New Zealand published also the highest number of articles per researcher (full-time equivalent FTE/1000) with R RES = 27.92, followed by Norway, South Africa, and Switzerland. Here the USA ranked 16th ( R RES = 9.22) and Germany 19th ( R RES = 7.83). Unfortunately, the data for Australia was not available.
Inclusion of climate change indices
Carbon dioxide emission.
The linkage of country-specific number of publications on climate change with the countries’ CO 2 emission shown in Table 4 discloses Sweden as the leading country ( R CO2 = 29.28), followed by Switzerland ( R CO2 = 28.10), Denmark ( R CO2 = 23.01), Norway ( R CO2 = 20.47) and New Zealand ( R CO2 = 14.52). In this analysis, the most publishing countries fell sharply behind. UK ranked 6 th ( R CO2 = 14.36), Germany 17th ( R CO2 = 4.05), and the USA 19th ( R CO2 = 14.52).
Global climate risk index
For reasons of comparison, reference is made here to the results of the Global Climate Risk Index (CRI) [ 9 ]: The average ranking shows Puerto Rico, Myanmar, and Haiti as the most affected countries, while the assessment for 2018 ranked Japan, the Philippines, and Germany as the most affected countries [ 9 ]. The figures for the average number of deaths per 100,000 inhabitants from 1999 to 2018 as a reference point put some small island developing states (SIDS), such as St. Kitts and Nevis, Tuvalu, Kiribati, Seychelles, Marshall Islands, and the Maldives in the first place. Armenia, Iceland, Singapore, and Qatar also held leading positions. (Fig. 7 a).
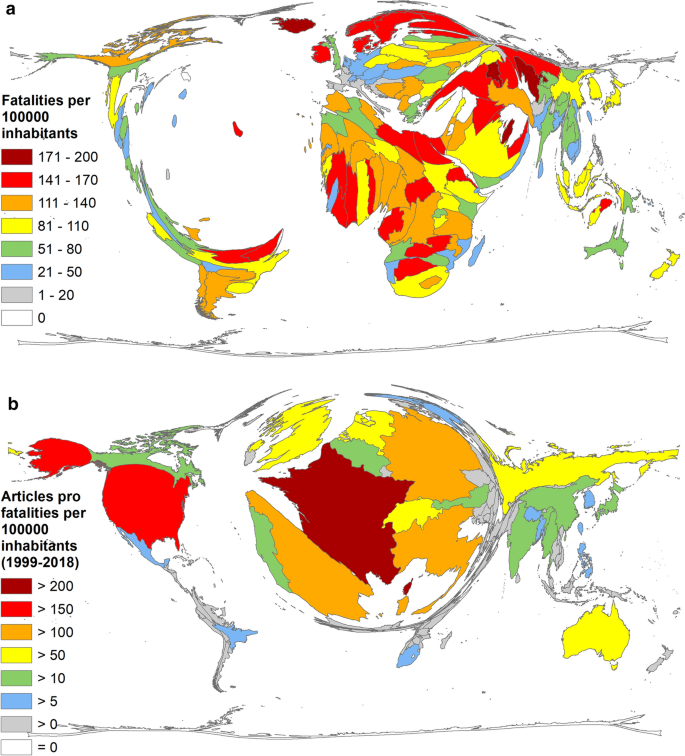
Global Climate Risk Index (1999–2018) [ 9 ]. a Average number of fatalities per 100,000 inhabitants. b Number of articles on climate change pro average number of fatalities per 100,000 inhabitants
The linkage of the number of publications on climate change to the expected increase in extreme events due to climate change discloses France as the leading country ( R CRI = 215.50), followed by the USA ( R CRI = 162.01), Spain ( R CRI = 145.80), Italy ( R CRI = 144.44), Germany ( R CRI = 140.78), and UK ( R CRI = 215.01). Of the countries most affected by climate change, Myanmar ranked 17th ( R CRI = 12.00), followed by Japan on rank 18 ( R CRI = 11.89), and the Philippines on rank 20 ( R CRI = 8.19) (Fig. 7 b). There was no correlation between the number of articles and the average number of fatalities per 100,000 inhabitants on average.
Sea-level rise
For reasons of comparison, reference is made here to the results of Kulp and Strauss [ 19 ]: According to their findings of the working group, China is by far the country with the highest number of people living on vulnerable land in million according to the CoastalDEM scenario (we here label it: P vul = 151.6) (Fig. 8 a). With P vul = 73, Bangladesh’s population is the second most affected, followed by India ( P vul = 151.6), Vietnam, and Indonesia ( P vul = 151.6). In addition to these absolute figures, the working group of Kulp and Strauss [ 19 ] put the number of affected people in relation to the total population. This results in a different picture (Fig. 8 b), with the small island states (Maldives, Marshall Islands, Tokelau, and Tuvalu) most affected, where more than 70% of the population will live on vulnerable land in 2100. In the South-American countries of Suriname and Guyana, more than 60% will live on vulnerable land, followed by Kiribati, Cayman Islands, and the Bahamas with more than 50% affected people. The Netherlands is the first European country in the ranking, where 55% of the inhabitants will be exposed to vulnerable land. The here determined most publishing countries on climate change, were following far behind: USA (2.3%), UK (9%), China (11%), Australia (4%), and Germany (2.5%) [ 19 ].
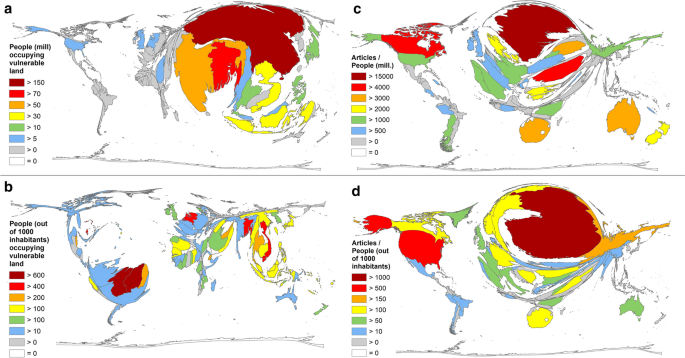
Estimated number of people exposed to vulnerable land in 2100 (CoastalDEM scenario: Sea Level Rise Modell K17, RCP 4.5, 95 percentile) [ 19 ]. a Number of people living on vulnerable land in mill. b Relative number of people (per 1000 inhabitants) living on vulnerable land. c Relation of the number of articles on climate change and the number of people living on vulnerable land in mill. High values of SIDS (Small Island Developing States) cannot be shown. The highest values have Maldives (87%), Marshall Island (85%), Tokelau (78%), Tuvalu (73%). d Relation of the number of articles on climate change and the relative number of people (per 1000 inhabitants) living on vulnerable land in mill
Here we have calculated the ratio of countries’ publication performance on climate change in relation to Kulp et al.’s absolute ( R absolute ) and relative figures ( R relative ) of Kulp and Strauss [ 19 ] (Fig. 7 c, d). In terms of the relation of articles on climate change to the absolute numbers, Sweden was leading ( R absolute = 15,187), followed by Canada ( R absolute = 4597), Romania ( R absolute = 4366), Australia ( R absolute = 3940), South Africa ( R absolute = 3054), and Lithuania ( R absolute = 3050). The most publishing countries ranked as follows: The USA ranked 11th ( R absolute = 1805), Germany 14th ( R absolute = 1619), and UK 17th ( R absolute = 986), while China followed far behind on rank 88 ( R absolute = 23).
The analysis of the relative ratios led to the following ranking: Finland ( R relative = 2123), USA ( R relative = 549), Russia ( R relative = 157), South Africa ( R relative = 150), Canada ( R relative = 149). In terms of most publishing countries, Germany was ranked 8th ( R relative = 130), UK 18th ( R relative = 61), and China 25th ( R relative = 32).
A significant correlation could be shown between the absolute numbers of people living on vulnerable land and the number of articles ( p < 0.001), while the relative numbers did not correlate with the number of articles ( p < 0.53).
Vulnerability and readiness
Correlation analysis of the two ND-GAIN indices (readiness and vulnerability) of 2017 and the number of articles were both significant ( p < 0.0001), but with different slopes. The correlation of the readiness index and the number of articles was significantly positive (Fig. 9 a), and the correlation of the vulnerability index and the number of articles was significantly negatively correlated ( p < 0.001) (Fig. 9 b).
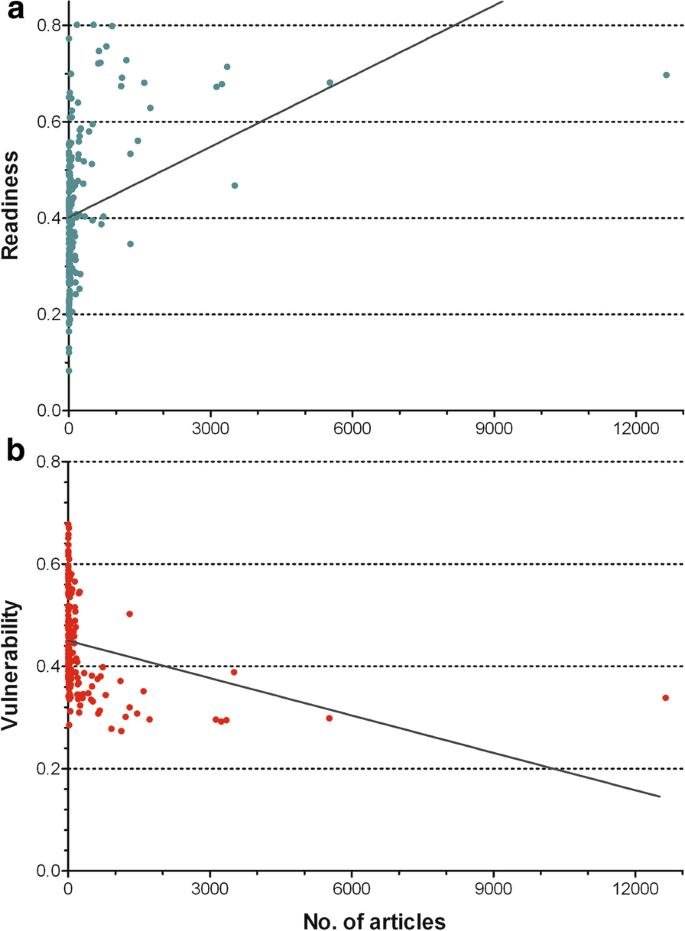
Correlation of the number of articles and indices of the ND-GAIN 2017 (Notre Dame Global Adaption Initiative) [27] regarding countries. a Readiness index, positive correlation ( p < 0.001). b Vulnerability index, negative correlation ( p < 0.001)
International networking
A total of n = 11,626 (29%) international cooperation articles were identified. Of these, n = 7995 were bilateral and n = 3165 trilateral collaborations, respectively. Four articles were worked out with at least 20 collaboration countries.
The first international cooperation in our database was published in 1975. Over time, the number of international partnerships increased exponentially, similar to the total number of articles, until it reached its maximum in 2014 with n = 1425 international collaboration articles.
The USA as core country of the international networking participated in the 5 strongest partnerships (Fig. 10 ): USA/UK ( n = 905), USA/China ( n = 830), USA/Canada ( n = 722), USA/Australia ( n = 563), and USA/Germany ( n = 534). Of the US articles, 37% were international collaborations, while more than half of the British articles and almost half of the Canadian and Australian articles were developed in international collaboration. Germany even conducted more than 60% of its studies with another country.
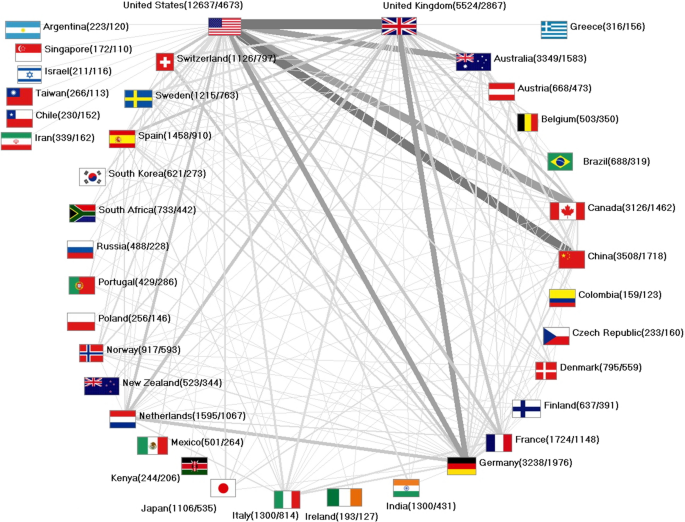
Network of internationally co-authored articles on climate change with numbers in brackets (number of articles/number of cooperation articles). The width of connecting lines represents the quantity of common articles (threshold: 40 collaboration articles between countries)
Progress of publications on climate change
The first article on climate change identified by our approach was published as early as 1910. It is an article published in Nature and asked the question of whether the Indian climate changed [ 20 ]. This early publication already addressed the causal link between climate change and anthropogenic influence. The author asked whether there are causal links of increased irrigation and forest loss, also in comparison to statements by Gilbert Walker, the General Director of Indian Observatories, who made connections between the air pressure in South America and the intensity of Monsoon in India, thus negating links between climate change in India and human interference.
In 1947, an English article raised the question of whether there was a connection between the retreat of glaciers and climate change [ 4 ].
In 1956, the carbon dioxide theory was confirmed by a US-American article, which referred to a series of articles published as early as the end of the nineteenth century [ 31 ]. The authors of these articles formulated the carbon dioxide theory and thus provided the most widely accepted explanation for the climate change already recognized at that time. However, this was later denied until it turned out to be true. In his study, Gilbert N. Plass from John Hopkins University has already seen the impact of human activities on the CO 2 balance through the combustion of fossil fuels, deforestation, and land management. In contrast to today’s threat awareness, the problem he discussed was the risk of new glacial formation due to the decrease of CO 2 caused by a changed balance in the atmosphere–ocean system [ 31 ].
A German article from 1961 also argues that "man-made effects on climate change “should not be underestimated as well as "the danger that such effects will work irreversibly against human benefit” [ 11 ].
A study on the astronomical theory , also known as the Milankovitch hypothesis of climate change, raised in 1969 the problem awareness of the scientific world with its Barbados data [ 23 ].
In 1988, the Intergovernmental Panel on Climate Change (IPCC), an intergovernmental body of the United Nations (UN), was established at the first world climate conference in Geneva with the aim to provide a wide range of scientific information on climate change to support governmental decisions [ 16 ]. As of this first world climate conference, the number of publications has risen firstly to a three-digit figure, which can also be seen from the sharp increase in relative numbers per 10,000 SCI articles.
Thereafter, the numbers increased steadily until 2003, when an exponential increase could be observed that was also reflected by the steep rise in relative numbers. At the COP in Milan (Italy) in 2003, all parties agreed to the Adaption Fund, which was primarily founded to support developing countries in their capacity to respond to the consequences of climate change. In the same year, an enormous heatwave caused many thousands of deaths in Europe [ 37 ]. Since European countries, in particular, are among the most publishing nations, this regional climate catastrophe has certainly contributed to a strong increase in research interest on climate change.
Also, in 2003, the most cited article of this study was published. By analyzing more than 1700 species, the meta-analysis of C. Parmesan and G. Yohe shows that biological trends are in line with predictions of climate change [ 30 ]. This successful publication certainly contributed to the fact that the highest average citation rate per year was achieved in 2003, initiating an exponential growth in publication output.
The citation numbers increased adequately to the publication numbers with some outstanding years, e.g., 1991, 2000, 2004, and 2010, latter the year with the highest number of citations so far. Many of the high impact articles are published in these years so that an association can be assumed.

Geographical aspects of publications on climate change
The USA, the UK, China, Australia, and Germany could be identified as the most publishing countries on climate change. This is not surprising, as it shows that mostly scientifically well-structured countries conduct most of the research, not only on climate change issues, as previous studies also have shown [ 17 ]. China, in particular, was catching up in the recent years due to its targeted research policy, which is represented by the enormous increase in expenditures on R&D [ 28 ].
The USA government, which is the most publishing country on climate change so far, is not exactly famous for its climate change–conscious attitude. The rejection of binding targets and the denial to sign the Paris Agreement confirms this. The USA is still the country with the highest expenditures on R&D and certainly one of the most preferred places to work for the most renowned scientists in the world. Despite the government’s attitude, its leading position in terms of publication output is not unique for climate change research and certainly not astonishing.
The results also show a clear dominance of European countries in the publication numbers on climate change. Also, Europe has a very good scientific infrastructure at its disposal. In contrast, most European countries signed up to the binding targets of the Paris Agreement to reduce emissions by at least 40% by 2030 compared to 1990 [ 10 ]. Denmark event targeted for a 70% reduction [ 7 ].
To evaluate the scientific landscape on climate change in greater depth, we extended the analyses to other, more differentiated parameters.
The Scandinavian countries have to be highlighted due to their leading position concerning various additional evaluation parameters, e.g., socioeconomic ratios. In general, Scandinavian countries have established good conditions for researchers and spend a lot on R&D. This is why research on climate change has also proven to be no exception. Sweden and Norway were leading in the analysis of their publication numbers in terms of national CO 2 emission, with Switzerland in between in 2nd place. This parameter had been chosen for the analysis in order to establish a link with countries’ obligations under the polluter-pays principle. In 2017, the highest emissions rates were released by China, USA, India, Russia, Japan, Germany, Iran, and Saudi Arabia. Sweden ranks first when putting the number of published articles in relation to the emission rate (threshold 300 articles), followed by Switzerland, Denmark, Norway, and New Zealand.
The Scandinavian countries are known to be “early adopters of renewable energy”. The share of renewable energy in Iceland is 77%, in Sweden 63%, in Norway 51% (despite the oil production capacity), in Finland 47%, and in Denmark 33%, in contrast to the EU28 with a proportion of only 21% [ 26 ]. Also, in terms of the relation to the number of people living on exposed land to sea-level rise, Sweden led in the evaluation of absolute numbers and Finland of relative numbers. With 1215 articles, Sweden ranked 12th regarding its absolute publication numbers, Norway 15th, Denmark 16th, and Finland 20th.
Switzerland, which is ranked second in terms of inclusion of CO 2 emission, is affected to a considerable extent by climate change due to its location in the European Alps and the progressive melting of glaciers and permafrost. Especially since tourism—above all skiing—is an important economic sector. Therefore, it is not astonishing that the focus of Swiss research is mainly on problems related to the Alpine region [ 6 ].
In terms of science-related parameters, such as GERD or number of researchers, both Australia and New Zealand came into focus. Since they are located close to each other, the intensity of cooperation in climate change research is understandable. The location near Antarctica on the one hand and the immense heatwaves with extraordinary effects on ecosystems and biosphere on the other hand form the background for relatively high investments in climate change research.
Looking at the average citation rate of the publishing countries ( n ≥ 30), Costa Rica occupied a prominent position. With 67 articles, Costa Rica is far behind in absolute terms. Nevertheless, these articles were cited 6291 times. Nearly half of the studies of Costa Rica are worked in collaboration with the USA. The Tropical Science Center (TSC) affiliated with the Monteverde Cloud Forest Biological Reserve in Costa Rica participated in a US-American and Costa Rican collaboration, with the 5th most cited article of this analysis, that deals with the impacts of climate change on wildlife [ 34 ]. The Center is also taking part in two other high-profile publications, which, like the most cited article, are also published in Nature . They all deal with the risk of extinction caused by global warming. Alan Pounds, biological scientist since 1996 at the TSC and focusing on the biological impact of climate change, found, e.g., a correlation between amphibian die-offs and rising average temperatures [ 32 ]. Previously, he worked at the Department of Zoology, University of Florida, USA, where he already collaborated with colleagues from Costa Rica. The pattern of the successful partnership of international networks can be seen in this example, which stands for mutual benefit for both cooperating countries.
In terms of citation rates, Estonia also took a leading position, as it is part of a Europe-wide meta-analysis on changes in phenology using data on more than 125,000 observational series of plants and animals to assess their response to climate change [ 22 ]. The resulting article, which was published in 2006 in Global Change Biology, received almost half of the Estonian citations.
The third country that should be highlighted regarding the citation rate of its articles is Iceland. Its articles were not counted among the high-impact publications. Instead, many of its articles achieved recognition with above-average citation rates. The location far in the north, close to Greenland and the Arctic Circle, is an advantage for all climate change projects that focus on melting glaciers in these regions, and the glacial retreat is here more and more evident. It has been assumed that all Islandic glaciers will be disappeared by the year 2200 [ 26 ]. Therefore, the most cited Icelandic article is the result of an international collaboration focusing on the regional differences in the last glacial period to better understand climate dynamics [ 3 ].
The comparison of the countries’ results in relation to the GDP put the insular state of Fiji, which consists of more than 300 islands, at the top of the evaluation. Currently, almost one million people are living in an area of about 18,000 square kilometers north of New Zealand in the South Pacific. Fiji has been selected to chair the 23rd climate summit 2017 in Germany. In the same year, the number of articles from Fiji reached its maximum, which seems to be associated. Nearly half of the articles are collaboration works with Australia. One of the advantages of research cooperation on climate change is the existence of Fiji’s coral reefs, their vulnerability, and their importance for coastal protection.
Worthy to note is also the rank of Denmark in terms of socioeconomic influence. In addition to Denmark’s otherwise equally good scientific infrastructure, its position in climate change research is certainly influenced by Greenland’s affiliation and the direct and immediate effects of climate change in this region located closest to the Arctic. The direct association to Greenland or the Arctic can be found in more than 200 Danish articles mostly focusing on Geoscience . The Niels Bohr Institute at the University of Copenhagen is leading in the climate change research based on ice cores. The ice core collection is considered as a “national treasure” and contains a deep drill core of more than 15 km in length [ 43 ].
In connection with the socioeconomic analysis, it is also remarkable that the African developing country Zimbabwe ranked 7th among the top 10. Unlike other African countries, it is relatively industrialized and produces twice the average amount of greenhouse gases [ 5 ]. Nevertheless, Zimbabwe—like other African countries—has to cope with droughts, freshwater and food shortages, diminished biodiversity, vector-borne diseases, and dry ups as a result of climate change. Zimbabwe was among the first countries to sign and ratify the UN Framework Convention on Climate Change (UNFCCC) in 1992 [ 29 , 41 ]. In 2011, it participated in the REDD+ program ( Reducing Emissions from Deforestation and Forest Degradation ), which aims to avoid 52 million tons of CO 2 over 30 years in Zimbabwe and in return to support the communities with financial aid for agriculture, fire prevention, and production methods to preserve forest areas [ 5 ].
France, which ranks 7th in terms of absolute publication numbers, led when the ratio between publication numbers and fatalities due to climate events of the CRI index is assessed. More than half of the articles on climate change are worked out with the participation of the French state research organization Centre National de la Recherche Scientifique (CNRS). The CNRS was ranked 4th by the Nature Index in 2017 regarding the largest contributors, behind the CAS (in this study identified as most publishing institute on climate change), Harvard University USA, and Max Planck Society Germany [ 25 ]. Plus, the majority of its articles is worked out as international collaboration (66.59%). The share of the other most publishing countries is considerably lower: USA (36.98%), UK (51.90%), China (48.97%), Australia (47.27%) Germany (61.10%), Canada (46.77%).
Nevertheless, the share of collaboration articles is relatively high in comparison to other research fields. This may be due to the majority of articles published after 2000, considering that the share of collaboration articles generally increases over time due to the international awareness of its benefits [ 1 ].
Articles on climate change focused on three main thematic groups, leading from the modeling of future scenarios to the environmental and socioeconomic impacts and the corresponding mitigation and adaptation measures. The readiness of countries and their vulnerability are inversely related to the number of articles published on climate change. Our results show the dominance of the Northern hemisphere in terms of publication output on climate change. Taking into account socioeconomic, research, and climate-specific characteristics, the order of the leading countries shifts, but the main actors remain the same with only a few exceptions. Only Costa Rica, Fiji, and Zimbabwe as developing countries came to play a role in the evaluation of the results. In principle, Africa, Asia, and South America are extremely under-represented. Many scientists are becoming aware of the advantages of international networking, which is of mutual benefit to all participating countries. However, particularly regarding climate change research, these benefits should be more frequently shared with developing countries, as the involvement of these most affected nations still is sparse.
In this context, the term “equity” is certainly familiar to all those interested in climate change research. There is a heated debate in the scientific community on whether scientific cooperation with developing countries should be called for. Many researchers see this as limiting the freedom of research. However, the principle of research responsibility should also be taken into account in this context. This should or must lead to a global risk-indexed joint planning because all scientists have only one planet to take care of. In this context, Prof. Drenth, Emeritus, Psychometrics and Organizational Psychology, Free University Amsterdam [ 8 ], asked the following questions for any scientist dealing with climate change: “Risks for whom? How far does the right to know go? What is the balance between self-determination and the interests of larger groups or the society as a whole? How certain does the scientist have to be before warning, especially in the case of irreversible developments?”.
The spread and economic impact of the current COVID 19 pandemic has reduced public and media interest in climate change issues. All the more reason to urgently press for the causes and consequences of climate change to once again become the focus of interest, while at the same time dealing with the consequences of the pandemic. Climate change must continue to be recognized as one of the most urgent global challenges. This makes it necessary to reconcile future scientific direction with the long-term environmental, social and economic consequences of the impacts of climate change that all countries are facing.
Availability of data and materials
The bibliometric data are the property of the Web of Science database and were obtained from it. Therefore, the authors are not allowed to pass on the data publicly or privately. Any researcher with access to the Web of Science database can obtain the data using the methods described in the paper. Readers who do not have access to Web of Science should contact Clarivate Analytics to obtain a license.
Adams J (2013) Collaborations: the fourth age of research. Nature 497:557–560. https://doi.org/10.1038/497557a
Article CAS Google Scholar
Aleixandre-Tudo JL, Bolanos-Pizarro M, Aleixandre JL, Aleixandre-Benavent R (2019) Current trends in scientific research on global warming: a bibliometric analysis. Int J Global Warm 17:142–169. https://doi.org/10.1504/Ijgw.2019.097858
Article Google Scholar
Blunier T et al (1998) Asynchrony of Antarctic and Greenland climate change during the last glacial period. Nature 394:739–743. https://doi.org/10.1038/29447
Bonacina LCW (1947) Climatic Change and the Retreat of Glaciers. 1 The Self-Generating or Automatic Process in Glaciation. Q J Roy Meteor Soc 73:85–000. https://doi.org/10.1002/qj.49707331506
Brazier A (2015) Konrad Adenauer stiftung, climate change in Zimbabwe, facts for planners and desicion makers. https://www.kas.de/c/document_library/get_file?uuid=6dfce726-fdd1-4f7b-72e7-e6c1ca9c9a95&groupId=252038 . Assessed Mar 2020
Bronnimann S et al (2014) Climate change in Switzerland: a review of physical, institutional, and political aspects. Wires Clim Change 5:461–481. https://doi.org/10.1002/wcc.280
ClimateHomeNews (2019) Demark adopts climate law to cut emissions 70% by 2030, Climate Home News. https://www.climatechangenews.com/2019/12/06/denmark-adopts-climate-law-cut-emissions-70-2030/ . Accessed Mar 2020 Climate Home News
Drenth P (2001) Freedom and responsibility in science: reconcilable objectives? Hamburg, 19–20 October 2001, Joachim Jungius-Gesellschaft der Wissenschaften, Symposium “Forschungsfreiheit und ihre ethische Grenzen”
Eckstein D, Hutfils M, Winges M (2019) Global Climate Risk Index 2019, GermanWatch. www.germanwatch.org/en/cri . Accessed Dec 2019
EU (2020) 2030 climate & energy framework. https://ec.europa.eu/clima/policies/strategies/2030_en . Accessed Mar 2020
Flohn H (1961) Mans activity as a factor in climatic change. Ann N Y Acad Sci 95:271. https://doi.org/10.1111/j.1749-6632.1961.tb50038.x
Gastner MT, Newman MEJ (2004) Diffusion-based method for producing density-equalizing maps. P Natl Acad Sci USA 101:7499–7504. https://doi.org/10.1073/pnas.0400280101
GermanWatch (2019) CCPI, Climate Change Performance Index. https://www.climate-change-performance-index.org . Accessed Dec 2019
Groneberg-Kloft B, Fischer TC, Quarcoo D, Scutaru C (2009) New quality and quantity indices in science (NewQIS): the study protocol of an international project. J Occup Med Toxicol 4:16. https://doi.org/10.1186/1745-6673-4-16
IPCC (2013) Climate Change 2013: The physical science basis. Contribution of working group i to the fifth assessment report of the intergovernmental panel on climate change. Cambridge University Press, Cambridge, United Kingdom and New York, NY, USA, p 1535
IPCC (2019) The Intergovernmental Panel on Climate Change. https://www.ipcc.ch . Accessed Dec 2019
Klingelhofer D, Braun M, Quarcoo D, Bruggmann D, Groneberg DA (2019) Research landscape of a global environmental challenge: microplastics. Water Res 170:115358. https://doi.org/10.1016/j.watres.2019.115358
Kopp RE et al (2017) Evolving understanding of Antarctic ice-sheet physics and ambiguity in probabilistic sea-level projections earths. Future 5:1217–1233. https://doi.org/10.1002/2017ef000663
Kulp SA, Strauss BH (2019) New elevation data triple estimates of global vulnerability to sea-level rise and coastal flooding. Nat Commun 10:4844. https://doi.org/10.1038/s41467-019-13552-0
Lockyer WJS (1910) Does the Indian climate change? Nature 84:178–178. https://doi.org/10.1038/084178a0
Magri M, Solari A (1996) The SCI Journal Citation Reports: A potential tool for studying journals? 1 Description of the JCR journal population based on the number of citations received, number of source items, impact factor, immediacy index and cited half-life. Scientometrics 35:93–117. https://doi.org/10.1007/Bf02018235
Menzel A et al (2006) European phenological response to climate change matches the warming pattern. Glob Change Biol 12:1969–1976. https://doi.org/10.1111/j.1365-2486.2006.01193.x
Mesolell KJ, Matthews RK, Broecker WS, Thurber DL (1969) Astronomical Theory of Climatic Change—Barbados Data J Geol 77:250. Doi: 10.1086/627434
Mills JN, Gage KL, Khan AS (2010) Potential influence of climate change on vector-borne and zoonotic diseases: a review and proposed research plan. Environ Health Perspect 118:1507–1514. https://doi.org/10.1289/ehp.0901389
NatureIndex (2018) 10 institutions that dominated science in 2017. https://www.natureindex.com/news-blog/twenty-eighteen-annual-tables-ten-institutions-that-dominated-sciences . Accessed Mar 2020
Naylor M (2019) How the Nordics are standing up to climate change STP. https://www.stptranscom/how-nordics-are-standing-up-to-climate-change/ . Assessed Mar 2020
ND-GAIN (2019) Notre Dame Global Adaptation Initiative, Country Index. https://gain.nd.edu/our-work/country-index/ . Assessed Dec 2019
OECD (2019) OECD Economic Surveys: China 2019. https://doi.org/10.1787/eco_surveys-chn-2019-en
OneYoungWorld (2020) The effects of climate change in Zimbabwe. https://www.oneyoungworld.com/blog/effects-climate-change-zimbabwe . Accessed Mar 2020
Parmesan C, Yohe G (2003) A globally coherent fingerprint of climate change impacts across natural systems. Nature 421:37–42. https://doi.org/10.1038/nature01286
Plass GN (1956) The carbon dioxide theory of climatic change. Tellus 8:140–154
Pounds JA et al (2006) Widespread amphibian extinctions from epidemic disease driven by global warming. Nature 439:161–167. https://doi.org/10.1038/nature04246
Ritchie H, Roser M (2019) Our world in data: CO 2 and greenhouse gas emissions. https://ourworldindata.org/co2-and-other-greenhouse-gas-emissions . Accessed Nov 2019
Root TL, Price JT, Hall KR, Schneider SH, Rosenzweig C, Pounds JA (2003) Fingerprints of global warming on wild animals and plants. Nature 421:57–60. https://doi.org/10.1038/nature01333
Sangam SL, Savitha KS (2019) Climate change and global warming: a scientometric study. Collnet J Scientomet 13:199–212. https://doi.org/10.1080/09737766.2019.1598001
Singh BB, Sharma R, Gill JP, Aulakh RS, Banga HS (2011) Climate change, zoonoses and India. Rev Sci Tech 30:779–788. https://doi.org/10.20506/rst.30.3.2073
Stott PA, Stone DA, Allen MR (2004) Human contribution to the European heatwave of 2003. Nature 432:610–614. https://doi.org/10.1038/nature03089
TheWorldBank (2019a) Data, GDP (current US$). https://data.worldbank.org/indicator/NY.GDP.MKTP.CD . Accessed Sept 2018
TheWorldBank (2019b) Data, Population, total. https://data.worldbank.org/indicator/SP.POP.TOTL . Accessed Sept 2018
UIS.Stat (2019) Data 2017. https://data.uis.unesco.org/Index.aspx . Accessed Nov 2019.
UNDP (2020) Climate Change Adaption, Zimbabwe. https://www.adaptation-undp.org/explore/eastern-africa/zimbabwe . Accessed Mar 2020
UNFCCC (2019) United Nations Climate Change, The Paris Agreement. https://unfccc.int/process-and-meetings/the-paris-agreement/the-paris-agreement . Accessed Dec 2019
UniversityCopenhagen (2020) New Ice Core Storage facility at the Niels Bohr Institute, University of Copenhagen. Official inauguration on 11 March. https://www.nbi.ku.dk/english/news/news20/new-ice-core-storage-facility-at-the-niels-bohr-institute-university-of-copenhagen.-official-inauguration-on-11-march/ . Accessed Mar 2020
van Eck NJ, Waltman L (2010) Software survey: VOSviewer, a computer program for bibliometric mapping. Scientometrics 84:523–538. https://doi.org/10.1007/s11192-009-0146-3
Watts N et al (2019) The 2019 report of The Lancet Countdown on health and climate change: ensuring that the health of a child born today is not defined by a changing climate. Lancet 394:1836–1878. https://doi.org/10.1016/S0140-6736(19)32596-6
Download references
Acknowledgements
Not applicable.
Open Access funding enabled and organized by Projekt DEAL. No funding has been received for this study.
Author information
Authors and affiliations.
Institute of Occupational, Social and Environmental Medicine, Goethe University, Theodor-Stern-Kai 7, 60590, Frankfurt, Germany
Doris Klingelhöfer, Ruth Müller, Markus Braun, Dörthe Brüggmann & David A. Groneberg
Unit Entomology, Institute of Tropical Medicine, Nationalestraat 155, 2000, Antwerp, Belgium
Ruth Müller
You can also search for this author in PubMed Google Scholar
Contributions
DK, DAG contributed to the development of the methodological platform. DK concepted, designed, drafted the initial manuscript, carried out the literature search and the analyses. DAG contributed to conception, design, and analyses. RM, MB, DB contributed to the literature search, interpretation, design of results. RM, MB corrected the draft. RM, MB, DB contributed with important expert content. All authors agree to be accountable for all aspects of the work. All authors read and approved the final manuscript.
Corresponding author
Correspondence to Doris Klingelhöfer .
Ethics declarations
Ethics approval and consent to participate, consent for publication, competing interests.
All authors declare that they have no competing interests.
Additional information
Publisher's note.
Springer Nature remains neutral with regard to jurisdictional claims in published maps and institutional affiliations.
Rights and permissions
Open Access This article is licensed under a Creative Commons Attribution 4.0 International License, which permits use, sharing, adaptation, distribution and reproduction in any medium or format, as long as you give appropriate credit to the original author(s) and the source, provide a link to the Creative Commons licence, and indicate if changes were made. The images or other third party material in this article are included in the article's Creative Commons licence, unless indicated otherwise in a credit line to the material. If material is not included in the article's Creative Commons licence and your intended use is not permitted by statutory regulation or exceeds the permitted use, you will need to obtain permission directly from the copyright holder. To view a copy of this licence, visit http://creativecommons.org/licenses/by/4.0/ .
Reprints and permissions
About this article
Cite this article.
Klingelhöfer, D., Müller, R., Braun, M. et al. Climate change: Does international research fulfill global demands and necessities?. Environ Sci Eur 32 , 137 (2020). https://doi.org/10.1186/s12302-020-00419-1
Download citation
Received : 25 June 2020
Accepted : 29 September 2020
Published : 15 October 2020
DOI : https://doi.org/10.1186/s12302-020-00419-1
Share this article
Anyone you share the following link with will be able to read this content:
Sorry, a shareable link is not currently available for this article.
Provided by the Springer Nature SharedIt content-sharing initiative
- Global warming
- Greenhouse effect
- Bibliometrics
- Socioeconomic indices
- Climate inequity
- Research investment
The Freezing-Thawing Index and Permafrost Extent in Pan-Arctic Experienced Significant Changes after the Global Warming Hiatus
34 Pages Posted: 15 Aug 2024 Publication Status: Under Review
HongXiang Guo
Beijing Normal University (BNU)
Wenquan Zhu
Cenliang zhao, liyuan chen.
Global warming has led to pronounced changes in pan-Arctic terrestrial environments. The temporal and spatial trends of ground surface freezing-thawing index (GFDD/GTDD) in pan-Arctic remain unclear. Additionally, the global warming trend continued to intensify after the end of global warming hiatus in 2013, but the changes of air surface freezing-thawing index (AFDD/ATDD) and permafrost extent in pan-Arctic in the decades preceding and following 2013 remain also unclear. In this study, the temporal and spatial trends of AFDD/ATDD and GFDD/GTDD in pan-Arctic (areas north of 45°N) from 2003 to 2023 were analyzed, with a particular focus on the changes in the decades preceding and following 2013. Then, the average permafrost extent in 2003 - 2013 and 2014 - 2023 were estimated based on the GFDD/GTDD, and changes in permafrost extent were analyzed. The results indicate that AFDD and GFDD show decreasing trends, and ATDD and GTDD show increasing trends, with the amplitudes of the AFDD/AFDD trends being greater than GFDD/GTDD. From 2003 to 2023, the AFDD decreased by 11.07 °C·days/a, the ATDD increased by 7.69 °C·days/a, the GFDD decreased by 5.74 °C·days/a, and the GTDD increased by 4.34°C·days/a. The fluctuations of FDD/TDD and permafrost areas in the decades preceding and following 2013 are significantly greater than the overall trends from 2003 to 2023. In 2014 – 2023 compared to 2003 – 2013, the average AFDD decreased by 150.29 °C·days (4.75%), the average ATDD increased by 70.44 °C·days (4.13%), the average GFDD decreased by 75.55 °C·days (4.9%), and the average GTDD increased by 38.5 °C·days (2.45%). The permafrost area decreased from 13.17 × 106 km2 in 2003 – 2013 to 12.35 × 106 km2 in 2014 – 2023, a reduction of 0.82 × 106 km2 (6.23%). The FDD decreasing trends and TDD increasing trends in Eurasia and Alaska have further intensified, with significant changes in the decades preceding and following 2013, leading to noticeable permafrost degradation. The permafrost southern boundary in Russia experienced the most significant degradation. In Alaska, the FDD increased and TDD decreased around 2020, leading to little changes in 2020 - 2023 compared to 2003 - 2005. In Canada, the FDD/TDD trends have decelerated from 2003 to 2023, and the permafrost area slightly increased.
Keywords: pan-Arctic, Freezing-thawing index, Permafrost extent, Spatial and temporal trends, Global warming hiatus
Suggested Citation: Suggested Citation
Beijing Normal University (BNU) ( email )
Wenquan zhu (contact author), do you have a job opening that you would like to promote on ssrn, paper statistics, related ejournals.
Subscribe to this free journal for more curated articles on this topic
Disentangling the complexities of how surface thermal factors influence the July precipitation in eastern China
- Journal of Climate In-Press

- ChangJiang Water Resources Commission

- Nanjing University of Information Science & Technology

- This person is not on ResearchGate, or hasn't claimed this research yet.
Discover the world's research
- 25+ million members
- 160+ million publication pages
- 2.3+ billion citations
No full-text available

To read the full-text of this research, you can request a copy directly from the authors.
- Recruit researchers
- Join for free
- Login Email Tip: Most researchers use their institutional email address as their ResearchGate login Password Forgot password? Keep me logged in Log in or Continue with Google Welcome back! Please log in. Email · Hint Tip: Most researchers use their institutional email address as their ResearchGate login Password Forgot password? Keep me logged in Log in or Continue with Google No account? Sign up

Maintenance work is planned from 21:00 BST on Sunday 18th August 2024 to 21:00 BST on Monday 19th August 2024, and on Thursday 29th August 2024 from 11:00 to 12:00 BST.
During this time the performance of our website may be affected - searches may run slowly, some pages may be temporarily unavailable, and you may be unable to log in or to access content. If this happens, please try refreshing your web browser or try waiting two to three minutes before trying again.
We apologise for any inconvenience this might cause and thank you for your patience.
Inorganic Chemistry Frontiers
Recent advances in the mechanism and catalyst design in the research of aprotic, photo-assisted, and solid-state li–co 2 batteries.

* Corresponding authors
a Centre for Hydrogenergy, College of Materials Science and Technology, Nanjing University of Aeronautics and Astronautics, Nanjing 210016, P. R. China E-mail: [email protected]
b Zhongyuan Critical Metals Laboratory, School of Materials Science and Engineering, Zhengzhou University, Zhengzhou, Henan 450001, P. R. China E-mail: [email protected]
c School of Materials Science and Engineering, Nanjing Institute of Technology, Nanjing 211167, P.R. China E-mail: [email protected]
d Graduate School of Advanced Science and Engineering, Waseda University, 2-8-26 Nishiwaseda, Shinjuku, Tokyo 169-0051, Japan E-mail: [email protected]
e Institut de recherches sur la catalyse et l'environnement de Lyon (IRCELYON), UMR5256, PRETTRE building, 2 avenue Albert Einstein, Villeurbanne cedex 69626, France
Carbon dioxide (CO 2 ), resulting from the combustion of fossil fuels, is widely recognized as one of the main contributors to global warming. However, the existing carbon capture methods lack stability and cost-effectiveness. In order to tackle this issue, lithium-carbon dioxide batteries (LCBs) have emerged as a promising solution due to their high energy density and environmentally friendly nature. Nevertheless, the development of LCBs is still in its early stages, facing challenges such as low catalyst efficiency, electrolyte system instability, and an unclear mechanism. This review focuses on the reaction mechanism and cathodic catalysts of various types of LCBs, which include aprotic LCBs, photo-assisted LCBs, and all-solid-state LCBs. Lastly, the review paper summarizes the current problems and challenges associated with LCBs, providing suggestions and solutions to further advance the development and research of the LCB system.
- This article is part of the themed collection: 2024 Inorganic Chemistry Frontiers Review-type Articles
Article information
Download citation, permissions.

H. Chen, X. Li, H. Xue, L. Jia, Y. Xu, Y. Tao, Y. Yan, X. Fan, J. He and T. Wang, Inorg. Chem. Front. , 2024, Advance Article , DOI: 10.1039/D4QI01570C
To request permission to reproduce material from this article, please go to the Copyright Clearance Center request page .
If you are an author contributing to an RSC publication, you do not need to request permission provided correct acknowledgement is given.
If you are the author of this article, you do not need to request permission to reproduce figures and diagrams provided correct acknowledgement is given. If you want to reproduce the whole article in a third-party publication (excluding your thesis/dissertation for which permission is not required) please go to the Copyright Clearance Center request page .
Read more about how to correctly acknowledge RSC content .
Social activity
Search articles by author.
This article has not yet been cited.
Advertisements

An official website of the United States government
The .gov means it’s official. Federal government websites often end in .gov or .mil. Before sharing sensitive information, make sure you’re on a federal government site.
The site is secure. The https:// ensures that you are connecting to the official website and that any information you provide is encrypted and transmitted securely.
- Publications
- Account settings
Preview improvements coming to the PMC website in October 2024. Learn More or Try it out now .
- Advanced Search
- Journal List
- v.7(11); 2021 Nov

Climate change/global warming/climate emergency versus general climate research: comparative bibliometric trends of publications
Rafael m. santos.
a School of Engineering, University of Guelph, Guelph, Ontario, N1G 2W1, Canada
Reza Bakhshoodeh
b Department of Civil, Environmental and Mining Engineering, University of Western Australia, Perth, 6009, Australia
Associated Data
Data will be made available on request.
This article presents and discusses the scientific publication record from 1910 to 2020 on two topics: "climate" (CL) and "climate change/global warming/climate emergency" (CC/GW/CE). The goal is to comparatively visualize how these two distinct publication records have evolved over time, from different classification perspectives, using publication ratios as the key indicator. It is found that research output related to the Earth's contemporary changing climate overtook that of general climate research in 2010, and the publication ratio (CC/GW/CE)/(CL) has been expanding in the last decade. There are significant differences in the publication countries and sources between the two topics. Differentiation factors that affect the level of research output and engagement on the climate challenge include island versus landlocked nations, specialized versus general scientific journals, academic versus institutional organizations. The future of the publication records is discussed, such as the emergence of new terms to refer to the climate challenge, such as “climate emergency”.
Bibliometric analysis; Scientometrics; Human influence on climate; Natural control of climate; Improving climate monitoring; Climate variability; Climate models; CO 2 .
1. Introduction
The climate of a region is its average or typical weather over a long period of time; for example, the climate of Antarctica is freezing cold, and Hawaii is warm and sunny. Climate change, therefore, is a long-term change in the typical or average weather of a region; in the last few decades, industrial and human activities have led to gradually accelerating changes in the climate, including an annually incremental increase in the average surface temperature, which has been defined as climate change ( IPCC, 2014 ). Climate change also has noticeable negative impacts on other parts of the planet, like changes in ecosystems and desertification, rise in sea level, flooding, and drought ( Hisano et al., 2018 ; Ouhamdouch et al., 2019 ). The Intergovernmental Panel on Climate Change (IPCC) defines climate change as “a change in the state of the climate that can be identified… by changes in the mean and/or the variability of its properties and that persists for an extended period” ( IPCC, 2018 ). Climate Change (CC), Global Warming (GW) and more recently Climate Emergency (CE) have been, in the past decade and longer, terms synonymous with the greatest sustainability challenge of the 21 st century ( Munasinghe, 2010 ; Kyte, 2014 ; Princiotta and Loughlin, 2014 ; Martens et al., 2016 ).
Climate change mitigation is a technological measure aiming to reduce the amount of anthropogenic emissions of greenhouse gases (GHG) ( Fawzy et al., 2020 ), and can be divided mainly into: (i) mitigation technologies, which focus on reducing fossil-based CO 2 emissions, including nuclear power, renewable energies, and carbon capture and storage ( Ricke et al., 2017 ; Bustreo et al., 2019 ); (ii) negative emissions technologies, which aim to capture and sequester atmospheric carbon to reduce carbon dioxide levels, and include approaches such as BECCS (bioenergy with carbon capture and storage), DACCS (direct air carbon capture and storage), enhanced rock weathering, and ocean fertilization ( Goglio et al., 2020 ; Khalidy and Santos, 2021 ; Lezaun, 2021 ); and (iii) geoengineering techniques that change the Earth's radiative energy budget to stabilize or reduce global temperatures, such as stratospheric aerosol injection, and marine cloud brightening ( Lockley et al., 2019 ; Osman et al., 2020 ). Controlling and reversing climate change is expected to be a major concern and undertaking for mankind in the forthcoming decades.
Bibliometric analysis is a popular technique commonly employed to investigate the internal relationships in the body of scientific outputs in the literature. This method is helpful for researchers who are interested in but unfamiliar with a specific field to understand the status of this field quickly. Various bibliometric studies have explored different topics related to climate change ( Demiroglu and Hall, 2020 ), global warming ( Marx et al., 2017 ), climate change's impact on human health, agriculture, and water resource management ( Wang et al., 2014 ; Janssen et al., 2006 ; Li et al., 2011 ; Wei et al., 2015 ), and various scientific ( Oliveira et al., 2020 ) and technological ( Sobreira et al., 2020 ) questions. Studies that merge traditional bibliometrics with scientific topics also term these types of studies scientometrics ( Janmaijaya et al., 2018 ; Sobreira et al., 2020 ). Such studies often rely on the most comprehensive literature databases available, including Web of Science and Scopus ( Sobreira et al., 2020 ; Salmerón-Manzano and Manzano-Agugliaro, 2017 ; Macchi Silva et al., 2019 ). It is also common for such studies to span several decades ( Janmaijaya et al., 2018 ; Oliveira et al., 2020 ), and cover regional ( Marx et al., 2017 ; Demiroglu and Hall, 2020 ) to global ( Salmerón-Manzano and Manzano-Agugliaro, 2017 ; Oliveira et al., 2020 ) topics.
In the last years, scientific publications and reports by scholars that study different aspects of climate change have rapidly increased ( Aleixandre-Benavent et al., 2017 ). Aleixandre-Benevant et al. (2017) evaluated that the number of publications increase by over six-fold between 2005 and 2014. They used social networks to conclude that the United States is at the center of much of the research, and found relationships between keywords to find to which fields of research climate change research is primarily interconnected with (main keywords were: CO 2 , adaptation, model, temperature, and impact). According to the results of Fu and Waltman (2021) , the number of publications on climate change topics in a country reflects the priorities set by its government to cover some of the existing issues. Geography and level of economic development were other factors associated with the scientific output of various countries or regions. Fu and Waltman (2021) also point to how the purpose of the research has been shifting in the last two decades from that concerned with the causes and effects of climate change to measures to reverse or incentivize the reversal of climate change. Due to the growing scientific and public attention to climate change, researchers have used the bibliometric method to characterize the intellectual landscape of climate change, including the impact of climate change on migration ( Milán-García et al., 2021 ), tourism ( Fang et al., 2018 ), and infectious diseases ( Li et al., 2020 ).
An important aspect of bibliometric studies is the choice of search string used to retrieve publications from databases ( Haunschild et al., 2016 ). Using too restrictive or specific keywords (e.g., simply “climate change”) can lead to an incomplete search record, so authors frequently use combinations and variations of keywords. For example: Aleixandre-Benevant et al. (2017) utilized [“climate change” OR “climate changes” OR “climatic change” OR “climatic changes”]; Fu and Waltman (2021) utilized [“climate chang∗” OR “climatic chang∗” OR “climate variabilit∗” OR “climatic variabilit∗” OR “global warming” OR “climate warming” OR “climatic warming”]; and Tan et al. (2021) utilized the largest combination among these three, [“climate change∗” OR “climatic change∗” OR “climatic variation” OR “climatic oscillation” OR “environmental risk∗” OR “environmental exposure” OR “environmental externalities” OR “ecological risk∗” OR “eco-risk∗” OR “climatic risk∗” OR “ecological management∗” OR “ecological governance” OR “ecological control” OR “environmental governance, environmental management∗” OR “environmental control” OR “environmental improvement” OR “eco-environmental risk∗” OR “low carbon” OR “carbon emission∗” OR “cost of emission reduction” OR “emission reducing potential” OR “emission reduction”]. Evidently, the search of Tan et al. (2021) would lead to inclusion of papers not related to climate change, such as those related to general climate research and those related to all forms of environmental impact and pollution. Fu and Waltman's search string also runs the risk of including general climate research via the term “climate variability”, but is the only one of the three to have included the term “global warming”. Haunschild et al. (2016) present a detailed discussed on how truncation and other operators can be used to narrowed down a bibliometric search to a specific area of research (climate change in their case), and also how additional keywords can then be used to split a large dataset into sub-sets based on specific sub-areas of the research field (e.g., the effects of climate change on ice and snow using the search terms [“∗ice∗” OR “∗glacier∗” OR “∗snow∗” OR “∗frost∗”] or on oceanic currents using the search terms [“∗el nino∗” OR “∗elnino∗” OR “∗southern oscillation∗” OR “∗enso∗” OR “∗Walker circulation∗” OR “∗north atlantic oscillation∗” OR “∗nao∗”]). Two things can be concluded from inspecting the various search strings used by authors of bibliometric studies: (i) it is critical to find a good balance between inclusion and exclusion of articles, and this is done by careful selection of search terms, focusing on the ones known to be frequently associated with the research topic, and by the use of the truncation (∗) operator; and (ii) there has not been a bibliometric study that has attempted to separate and analyze the unique research records related to climate change research from those related to general climate research. These are two important motivators on our present work.
In this article, we aim to comparatively explore the bibliometric and scientometric data on two topics: general “climate” research and “climate change/global warming/climate emergency” research. The former relates to research that builds on our understanding of what naturally governs the Earth's climate, and how the climate regulates natural processes on the Earth's surface; the latter relates to research that investigates what is causing the Earth's climate to change rapidly, primarily as a result of anthropogenic drivers, and what effects climate change has on the Earth's systems, and what could be done to mitigate or adapt to this. An inspiration we have used for this work is the historical importance of the work of British engineer Guy Callendar, who in 1938 pointed to the anthropogenic contribution to global temperature rise ( Callendar, 1938 ), at a time before climate change research took off. That is, climate change research originated from general climate research, and at some point in the 20 th century, as will be presented later on in this article, became a unique field of research with a unique publication record.
Apart from the novel comparative topical theme, another differentiator of this article is its use of publication ratio values. We define the publication ratios as the number of publications in a category in one record over that in another record, which help us to distinguish and contrast CC/GW/CE versus general climate (CL) research. This approach differs from other comparative studies (e.g., Baek et al. (2020) , Arana Barbier (2020) , Wang et al. (2021) ), in that the traditional approach for comparing records is to plot or tabulate the data of each record separately, and then compare the trends seen in each record. The publication ratio method allows more direct and precise comparisons, as are shown in this article. Yet another differentiator is that this article is hypothesis-driven; that is, hypotheses (presented below) are posed to guide the collection and analysis of the bibliometric and scientometric data. The testing of hypotheses allows for evaluation of the quality and effectiveness of the data analysis performed, and thus acts as a verification mechanism that often is lacking in traditional literature reviews. past studies on climate change do not attempt to isolate or exclude papers that relate to general climate research. To this end, we hereafter explore the publication trends of two records (CL and CC/GW/CE), since the topical terms appeared in the journal records in the early part of the 20 th century, to test the following hypotheses:
- 1. It is possible to substantially distinguish the scientific literature that pertains to the study of the aforementioned climate challenge (or solutions for mitigating it) from studies that address gaining a better understanding of the earth's climate itself, using topical keyword searches.
- 2. The scientific literature has become so enriched in works addressing the climate challenge that it surpassed climate research in terms of the number of publications sometime in the late part of the 20 th century.
- 3. The scientific literature that pertains to the climate challenge is at least partly distinct from that on climate research in terms of the venue of publication, country of origin of studies, and organizations that have conducted these works.
The present study is global in scope and covers a century of data, as it looks to highlight key moments in the publication record and scientific advancement histories, in addition to the temporal and various categorical trends. The following research questions have been formulated to contrast CL research versus CC/GW/CE research via hypothesis testing: (i) what are the dynamics of the conceptual structure of CC/GW/CE versus CL research; (ii) when the scientific record has become more enriched in CC/GW/CE versus CL research; (iii) in which countries the climate challenge has become the dominant topic and are there any relationships between countries and the dominant scientific topic?
2. Methodology
Web of Science (WoS) was used to search the scientific literature and collect the relevant publication data for analysis. The searches were conducted on August 7 th , 2021 (for 1900 to complete 2020 data); all data were collected within a short time on those days to obtain a snapshot of the publication record. Figure 1 shows the protocol used for this bibliometric study, which is classified into five steps detailed below.

Protocol used for the bibliometric study.
Step one: The search used a time span of 1900–2020 and all indexes within the Web of Science Core Collection, namely: Science Citation Index Expanded: SCI-EXPANDED (1900–2020), Social Sciences Citation Index: SSCI (1900–2020), Arts & Humanities Citation Index: A&HCI (1975–2020), Conference Proceedings Citation Index - Science: CPCI–S (1990–2020), Conference Proceedings Citation Index - Social Science & Humanities: CPCI-SSH (1990–2020), and Emerging Sources Citation Index: ESCI (2015–2020). The two search strings used were: (i) TOPIC: ("climat∗ chang∗" OR "global warming∗" OR "climat∗ emergenc∗"); and (ii) TOPIC: ("climate" NOT ("climat∗ chang∗" OR "global warming∗" OR "climat∗ emergenc∗")). The former search string was used to collect papers related to research on the climate challenge (CC/GW/CE), and the latter search string was used to collect papers related to general research on the Earth's climate (CL). That is, these search strings tested Hypothesis 1.
Step two: In the results section, the document type was refined to ‘Article’. These searches yield 245,391 on the CC/GW/CE topic and 228,280 papers on the CL topic. The use of the NOR logical operator in the CL search string ensures that the two records are unique; that is, there are no repeating papers.
To verify if the search string used for CC/GW/CE research may have missed a substantial portion of research papers that did not use the three searched keywords, a third search was conducted using the following search string: TOPIC: ((("greenhouse gas∗" OR "GHG∗") NOT ("climate" OR "climate change" OR "global warming" OR "climate emergency"))). This search yielded 29,943 articles. This represents 11.56% of articles obtained with the combined search strings. In the Discussion and Conclusions section, the omission of these articles from the data analysis is explained.
Step three: The search results were at first analyzed using the Analyze Results feature of WoS. On the analysis page, it is possible to download tab-delimited text files containing a set of publication data according to the WoS category selected. Data files were obtained for the following four categories: publication years; organizations-enhanced; source titles; countries/regions. The data from these text files were then imported into Microsoft Excel for further processing and analysis. These data and analyses enabled testing Hypotheses 2 and 3. More details on the data handling procedure are provided in the Data Analysis section.
One additional procedure used was to recover keywords from the search records. This was done using the Export feature of WoS on the search results page to generate Excel files containing various attributes of each paper, including the keywords. Keywords were compiled from the top 100 cited papers from both topics (CC/GW/CE and CL) as of August 7 th , 2021. These keywords were used to generate word clouds using the software Wordle ( Feinberg, 2020 ).
Step four: A bibliometric analysis including keywords co-occurrence, countries collaboration, most relevant words, beamplots and affiliations was also performed on the full search results, which was exported from WoS as a bibtex or CSV file, using the bibliometrix package ( Aria and Cuccurullo, 2017 ) in RStudio software Version 1.2.5001 ( R Core Team, 2019 ). The bibliometrix R-package provides a set of tools for quantitative research in bibliometrics and scientometrics. It is written in the R language ( Ihaka, 1998 ), which is an open-source environment and ecosystem ( Aria and Cuccurullo, 2017 ). The codes used in this paper are provided at the end of the Supplementary Materials. Beamplot percentile data was obtained from author profiles found within Web of Science.
These data and analyses enabled testing Hypotheses 2 and 3, which are shown in the Supplementary Materials. This package uses the meta-data in the Web of Science citations to calculate and rank country production, journal sources, and country collaborations.
Step five: The previous steps (step one to four) were for all selected databases. In this step, each database was separately selected for analysis. Therefore, steps 2 to 4 were repeated again, and the results from each search were imported to Excel and Rstudio for further analysis.
3. Publication record highlights
From 1910 to 1970, the publications record for CC/GW/CE shows only eight entries, with 1971 being the first year with multiple (three) records. In fact, a line can be drawn at 1970 with the publication of Berton's paper titled “Carbon dioxide and its role in climate change” ( Benton, 1970 ). This is the first of the papers on record to specifically address contemporary anthropogenic climate change. It does not mean that only in 1970 the role of humans on climate change was understood; such hypothesis dates to decades earlier. But it may be one of the first papers to consistently use the term “climate change” to describe the observed phenomena (increasing atmospheric concentration of greenhouse gases and increasing global surface temperatures, as discussed in the paper). Another evidence of this shift in terminology is that two of the three 1971 papers, those by Frisken (1971) and Kopec (1971) , address the pressing climate challenge. This is in contrast with the first paper on this record, the 1910 Nature article by Lockyer titled “Does the Indian climate change?” ( Lockyer, 1910 ), which discusses short term observations of changing frequency of monsoons to conclude that the climate on the sub-continent varies from year to year, with both short- and long-term trends, but the influence of humans on these trends is not addressed, in fact, the opposite (the natural control of climate) is inferred. Notably, both Frisken (1971) and Kopec (1971) highlight that by the early 1970's it is well accepted that humans can have a significant effect on the climate by air emissions, but that at that time (when CO 2 atmospheric concentration have just surpassed 320 ppm ( Benton, 1970 )), the role of nature was still deemed stronger than the role of humans.
One way to contrast the publication record of CC/GW/CE versus that of CL is to compare their most cited works. Tables S-1 and S-2 in the Supplementary Materials also present the classification of 50 top cited papers for each record. Briefly reviewing these papers (i.e., reading the paper's aims and conclusions) helps understanding if the two data records contain the required topical selection of CC/GW/CE versus CL. Based on the results from these tables, for CC/GW/CE records, 45 papers are correctly classified, two are unusually misclassified, and three of them are outliers. While for CL records, 41 papers are correctly classified in CL subject, eight are misclassified, and one is an outlier. Within the top 5 most cited papers of each record, seven of the ten papers are correctly classified, two are unusually misclassified, and one is an outlier, as follows. Table 1 presents the publication history of the first authors of the five most cited journal articles from each record (according to Tables S-1 and S-2), in terms of the three most cited papers authored or co-authored by these first authors, on any topic (in some cases, the same topic as the record, and in some cases on other topics). Notable, the first most cited paper of each of these authors is also their highly cited paper according to Tables S-1 and S-2.
Table 1
The first three most cited journal articles of the five first authors of the highest cited papers from the two records (CC/GW/CE and CL), as tabulated in Tables S-1 and S-2.
Rayner, Nick | 1985 | ( ) | 6,427 | Article | CC | ( ) | 5,610 | Review | CL | ( ) | 3,208 | CL | Article |
Parmesan, Camille | 1987 | ( ) | 6,216 | Article | CC | ( ) | 6,071 | Review | CC | ( ) | 4,964 | CC | Review |
Kottek, Markus | 2005 | ( ) | 4,896 | Article | CL | ( ) | 509 | Article | CC | ( ) | 48 | CL | Editorial Material |
Thomas,Chris D. | 1984 | ( ) | 4,324 | Article | CC | ( ) | 2,375 | Article | CC | ( ) | 1,643 | CC | Article |
Allen, Craig D. | 1994 | ( ) | 3,731 | Article | CC | ( ) | 2,153 | Review | CC | ( ) | 1,366 | CC | Article |
Kalnay, Eugenia | 1976 | ( ) | 21,389 | Article | CL | ( ) | 3,316 | Article | CL | ( ) | 2,349 | CL | Artile |
Hijmans, Robert J. | 1996 | ( ) | 12,994 | Article | CL | ( ) | 5,047 | Article | CL | ( ) | 2,089 | CL | Article |
Taylor, Karl E | 1976 | ( ) | 8,578 | Article | CC | ( ) | 3,495 | Article | Out of scope | ( ) | 2,059 | CC | Article |
Phillips, Steven J. | 1969 | ( ) | 8,303 | Article | CL | ( ) | 5,047 | Article | CL | ( ) | 3,375 | Out of scope | Article |
Tenenbaum, Joshua B. | 1991 | ( ) | 7,281 | Report | Out of scope | ( ) | 654 | Review | Out of scope | ( ) | 620 | Out of scope | Article |
Rayner et al. (2003) present sea ice and sea surface temperature and nighttime marine air temperature data sets, starting from 1871. That is, the study covers parameters pertinent for climate change research and the contemporary post-industrial revolution period attributed to anthropogenic climate change; after 18 years since their first publication in 1985, the first author reached their most cited paper in 2003. Parmesan and Yohe (2003) showed that climate change effects on living systems could be discerned from non-climatic effects by looking for systematic trends over diverse species and geographic regions; after 16 years since their first publication in 1987, the first author had reached their most cited paper in 2003. Kottek et al. (2006) provide a climate classification map update valid for the second half of the 20 th century, which was updated from the original 1961 Wladimir Köppen map. One motivation for this update was that climate changes have occurred and thus up-to-date global temperature and precipitation data sets were required to update the geographical distribution of the various climate zones (equatorial, arid, warm temperate, snow, and polar, and the various sub-classifications); one year after the first author's first publication on 2005, they reached their most cited paper in 2006. Thomas et al. (2004) showed how climate change leads to species-level extinction. They concluded that 18%–35% of species would be committed to extinction by 2050 because of climate change, in part because of habit loss due to changes in biome; after 20 years since the first author's first publication in 1984, they had reached their most cited paper in 2004. Allen et al. (2010) studied the effect of climate change and drought on trees mortality risks. They concluded that there is a direct relationship between tree mortality rates and heat severity and climate change; after 16 years since the first author's first publication in 1994, they had reached their most cited paper in 2010. All of these five highly cited papers are thus correctly classified under the CC/GW/CE topic. In addition, most (7 out of 10) of their second and third most cited papers are in the same research area (CC/GW/CE) as the record.
Kalnay et al. (1996) investigated how improvements to climate monitoring can avoid misinterpretation of climate variations that are not a result of climate change. The study is not concerned about studying climate change directly, even if the advances can benefit climate change research, and after 20 years since the first author's first publication in 1976, they had reached their most cited paper in 1996. Hijmans et al. (2005) developed a method for very high-resolution interpolation of temperature and precipitation climate data, which can be used to generate accurate climate surfaces (i.e., continuous grids); and after nine years since the first author's first publication in 1996, they had reached their most cited paper in 2005. This advance can help improve the analysis of climate change since more accurate values are obtained, though this was not the main aim of the study. An example was provided on how for Madagascar, the newly interpolated data set does not show direct evidence of climate change between 1930 and 1990. Another example stated that an insufficiently dense station network could lead to erroneous climate change conclusions. Phillips et al. (2006) present a model of the distribution of biological species due to geographic distribution, including climatic variables and conditions. The model was posed as being able to predict the movement of species due to climate change, such as invasive species, but this was not the study's main aim; after 37 years since the first author's first publication in 1969, they had reached their most cited paper in 2006. These are the three out of five highly cited papers correctly classified under CL. In addition, nearly all (5 out of 6) of their second, third most cited papers are also classified as CL research.
Tenenbaum et al. (2000) is the outlier. This paper does have relevance for CL research, as it pertains to the development of nonlinear algorithms to find trends in complex and large data sets, such as climate data sets, and is certainly not about CC/GW/CE. So while correctly classified, due to the use of the word “climate” in the abstract, the paper's topic is largely mathematical rather than about natural or engineering sciences. Taylor et al. (2012) is the paper that was unusually misclassified. This article does not have an abstract registered in WoS, and the article's single keyword registered in WoS is "climate" (the article itself does not have a keywords list). The article is in fact, about CC/GW/CE research; thus, the unusually incomplete record for this article caused it to be misclassified. These two papers highlight that the CL record is less robust than the CC/GW/CE record, particularly because of the CL record's less specific search string. While a weakness, the more analytical data processing presented in the Data Analysis section will show that this record is still useful for contrasting against the CC/GW/CE to yield dataset level (as opposed to paper-by-paper) trends and conclusions.
Table S-3 in the Supplementary Materials shows the top 5 cited paper in both records, which highlighted in blue (similar to the first column of Table 1 ) and 5 top papers in terms of citations which have cited these papers which are highlighted in grey. Almost all papers in each row are following the main papers’ topics (paper in the first column). For example, all articles that cited Hijmans et al. (2005) and Kalnay et al. (1996) were about climate modelling. Table S-4 in the Supplementary Materials lists the top 5 hot papers in both records which are highlighted in blue, and the top 5 hot papers in terms of citations that have cited the paper in the first column, which are highlighted in grey. According to WoS, hot papers are those that have been published in the last two years and have received enough citations to place them in the top 0.1% of papers in their academic fields. These papers demonstrate potential research hotspots and future research directions, providing readers with a more comprehensive understanding of these two studies. COVID-19 topics are one of the hottest topics due to the current situation and pandemic that most countries are dealing with; these papers cover the impact of COVID-19 on various aspects of our climate such as air pollution and microplastics. Furthermore, the majority of COVID-19-related papers are about climate change, which is classified in the CC/GW/CE record. Other hot topics in both records include air quality and wildlife conservation, such as insect extinction.
Bornmann and Marx first introduced beamplots in 2014 to better visualize the citation impact and productivity of researchers. In addition, beamplots are used to see performance variation over time to make more informed decisions about research impact and evaluation ( Bornmann and Marx, 2014 ). The beamplot represents a single frame of an author's output (the citation performance of an author's entire publication list), which reflects how it varies over time. In the beamplot, each dot represents a specific publication and its position is based on its publication year and its normalized citation percentile score (0–100). For example, a score of 90 for an article means that the article is among the top 10% most cited publications of the subject area, document type, and year.
For the first authors of the top 3 most cited papers in each record, as listed in Table 1 , the citation percentiles of their first authors were higher after publishing these articles, except in the case of Camile Parmesan, first author of Parmesan and Yohe (2003) . Figure S-1 in the Supplementary Materials shows the beamplots of these six authors from both records. For example, in the case of N.A. Rayner, who has published the highest cited paper in the CC/GW/CE record, the mean citation percentile of their papers published after their highest cited paper ( Rayner et al., 2003 ) has increased from 58% to 80%. Likewise but to a much lesser extent, for Eugenia Kalnay, who has published the highest cited paper in the CL record, the citation percentile was increased from 64.7% to 65.3%. This indicates that these authors either had more impactful research output following the publication of their most cited paper, or became better or more widely known after that date and hence received more citations to their latter work than their earlier work. The citation percentile of Camille Parmesan, who has the second highest cited paper ( Parmesan and Yohe, 2003 ) in the CC/GW/CE record, was lower after 2003, when they published their highest cited paper, than before 2003. Figure S-1 shows that their citation percentiles during 2009 and 2010 were zero, which were for five book chapters, and causes this difference between the citation percentile before and after publishing their highest cited paper in 2003. Excluding these book chapters from the beamplot analysis leads to the conclusion that the author's performance actually improved after 2003. In summary, it is commonly the case that highly cited papers, whether they be on CC/GW/CE or CL topics, typically boost an author's citation profile.
Figures 2 and and3 3 present the word clouds generated for the keywords extracted from the top 100 most cited papers in each record. In contrast to the aforementioned analysis of the top 5 most cited papers in each record, which showed significant differences in the two records, the word clouds are qualitatively less precise. Table 2 also presents the top 10 words frequency for the top 100 cited papers in each record. It is understandable that research on CC/GW/CE will use many similar keywords to more general research on the Earth's climate, thus several terms are similarly enlarged on both clouds. For example, model, variability, temperature, precipitation and circulation are some of the main words on both clouds. In fact, the vast majority of words from Figure 2 also appear in Figure 3 , even if in a different size. Climate change and CO 2 are the two terms in Figure 2 that are particularly distinct from Figure 3 , which is expected given that these are key topics of CC/GW/CE research. Figure S-2 in the Supplementary Materials also shows the word dynamic of both records over time. Based on the results from this figure, “climate change” and “climate” terms had the highest increase over time in terms of occurrence in articles. The conclusion from word clouds is that they are visually interesting, but are not ideal tools to evaluate two unique but topically similar publication records. As aforementioned, the Data Analysis section presents more deeply analytical comparisons between the two records, from which clearer trends can be seen.

Word cloud of keywords from top 100 most cited papers on CC/GW/CE research.

Word cloud of keywords from top 100 most cited papers on CL research.
Table 2
Word frequency of top ten used words in Figures 2 and and3 3 .
CC/GW/CE record | CL record | ||
---|---|---|---|
Word | Frequency ↓ | Word | Frequency ↓ |
climate-change | 140 | climate | 129 |
temperature | 55 | variability | 51 |
variability | 47 | model | 50 |
trends | 46 | temperature | 31 |
climate | 36 | ocean | 22 |
model | 35 | parameterization | 22 |
united-states | 33 | precipitation | 22 |
co2 | 26 | vegetation | 20 |
dynamics | 25 | sensitivity | 19 |
impact | 24 | dynamics | 18 |
Figure S-3 in the Supplementary Materials shows the co-occurrence analysis of keywords using the bibliometrix package in Rstudio in order to find research focus ( Aria and Cuccurullo, 2017 ). Based on results from this figure, “climate change”, “climate”, and “variability” were the most frequent words among all keywords from 500 top most cited papers, which were similar to the key topics from the word clouds (Figures 2 and and3 3 ).
4. Data analysis
This section is sub-divided into the four categories of data collection and analysis of the publication records: (i) year of publication; (ii) country (corresponding author's) of publication; (iii) source (i.e., journal) of publication; and (iv) organization (corresponding author's) of publication.
4.1. Year of publication
Figure 4 and Table S-5 in the Supplementary Materials present the data analysis for the year of publication, ranging from 1910 to 2020. The number of articles published per year in the two publication records (CC/GW/CE and CL) was compiled from WoS. For each year, a ratio of the number of articles in the CC/GW/CE record over the number of articles in the CL record was calculated. This ratio is plotted as a function of time in Figure 3 a. The purpose of this ratio is to help visualize when the scientific record became more enriched in CC/GW/CE versus general CL research; that is when the ratio surpasses a value of one. This occurred in 2010, and the ratio has since increased to 1.36 in 2019 and then to 1.45 in 2020 (a full-year record). Notably, before 1989, the ratio was consistently smaller than 0.1, meaning that CC/GW/CE research was scarce for much of the 20 th century. The exceptions in 1910, 1939 and 1941 are due to the very small number of CL publications on record for those decades. From 1989 onwards, the ratio increases nearly every year (in fact, it increases 28 out of 31 times, and every year since 1997).

Data for CC/GW/CE and CL records for year of publication: a) Publication ratio ((CC/GW/CE)/(CL)) as a function of time (years); b) Number of publications per year in the CC/GW/CE record versus those in the CL record (dashed line illustrates the 1:1 mark), which are labelled with the year of each ratio for the period 2006 to 2020 (over this recent period, the annual CL publications continuously increased).
The number of publications in both records rose by orders of magnitude over the last several decades, and Figure 4 b helps to visualize this climb. The CL record crossed 100 publications per year in 1975 versus 1990 for the CC/GW/CE record. The CL record crossed 1,000 publication per year also first, in 1991, followed by the CC/GW/CE record in 1996. Then both records breached 10,000 articles in a year in 2012. This coincides almost exactly with the 2010 threshold when the CC/GW/CE record overtook the CL in the number of publications per year. Points on Figure 4 b above the dashed line indicate the records from the last decade, while those below the dashed line correspond to the pre-2010 record.
Figure 5 breaks down the two data records for the number of articles per year ranging from 1910 to 2020 according to the databases that make up the Web of Science Core Collection. The purpose of this analysis is to visualize if any unusual or sudden changes in the underlying databases could contribute to the trends observed in the full data sets. This could include the effect of databases entering the coverage of the Core Collection in a particular year, or the databases changing their coverage at some point in time. Figure 5 shows that the two largest and oldest databases, namely SCI-EXPANDED and SSCI (with coverage commencing in 1900), have similar temporal trends, to each other and to the Core Collection, given that they make up the most substantial portion of the latter. The ESCI is a newer database (started in 2015), and the A&HCI is a database with a focus on research areas far from the theme of climate research, hence the smaller size of its records in this analysis; their data set trends also are also in overall agreement. Figure 5 is plotted on a log-scale to magnify trends of the smaller data sets, and variability in the two CPCI data sets is evident for both records. Likely this variability is at least partly related with variable number of conference proceedings being indexed by Web of Science each year, and underlying changes in the types of venues used for publication of peer-reviewed papers. Notwithstanding, the small numbers of these data sets (in the order of tens to hundreds of papers per year in the last decades) have insignificant impact on the trends of the much larger overall Core Collection data sets. Tables S-6 and S-7 in the Supplementary Materials show the number of articles in the CL and CC/GW/CE records per year for all databases.

Data for the number of articles per year for all databases in logarithmic scale: a) CC/GW/CE and b) CL.
4.2. Country/region of publication
Figure 6 , Figures S-4 and S-5 in the Supplementary Materials, and Table S-8 in the Supplementary Materials present the data analysis for the country (or region) of publication for the full records ranging from 1910 to 2020. The number of articles published per country/region in the two publication records (CC/GW/CE and CL), from 1910 to 2020, was compiled from WoS. For each country/region, a ratio of the number of articles in the CC/GW/CE record over the number of articles in the CL record was calculated. This ratio is plotted for each country/region in Figure S-4, ordered from largest to smallest ratio. Countries/regions with a ratio greater than one have been more engaged in CC/GW/CE research, while those with a ratio smaller than one have been more engaged in CL research. The number of countries/regions for which a ratio was calculated is 210. An additional 32 countries of regions did not have a ratio calculated, either due to no CC/GW/CE or CL articles on record (this occurs for small states such as Equatorial Guinea and Turks and Caicos). In addition, countries that no longer exist and became part of other countries were merged with their successor countries which include the Soviet Union with Russia; Western Germany with Germany; Serbia Montenegro with Serbia; Yugoslavia with Serbia; Czechoslovakia with the Czech Republic; and Swaziland with Eswatini. The number of studies in these countries before and after merging is shown in Table S-7 in the Supplementary Materials. Figure S-4 shows that slightly more than half of the countries/regions have a ratio greater than one, indicating that the climate challenge has become a dominant scientific topic in many parts of the world. It is notable that the majority of countries/regions with ratios greater than 2 are island states, such as Philippines (ratio = 2.21), Fiji (ratio = 2.92), Bahamas (ratio = 3.23), Palau (ratio = 6.25), Micronesia (ratio = 11), and Kiribati (ratio = 11). This highlights that small island states are at most risk of the catastrophic effects of climate change, particularly rising sea levels ( Vitousek et al., 2017 ; Horton et al., 2014 ; Nunn, 2009 ; King and Harrington, 2018 ; Widlansky et al., 2015 ).

Data for CC/GW/CE and CL records for country/region of publication: Number of publications per country/region in the CC/GW/CE record versus those in the CL record (dashed line illustrates the 1:1 mark), which are labeled with the names of outlier countries.
In contrast, countries and regions with a ratio lower than 0.50 tend to be those of lower gross domestic product, those in arid regions of the world, or those landlocked nations, such as Albania, Djibouti, Algeria and Turkmenistan. Other countries of interest to view ratios for are those with long publication history (USA (0.91), England (1.17), France (0.86), Germany (0.92)) and the emerging/fast growing economies (China (1.07), India (1.00), Brazil (0.76), South Africa (1.27)). The average ratio of these eight countries is very close to 1 (0.99), showing that such countries contribute with diverse research. On a case-by-case basis, it may be possible to claim which countries are more engaged in the climate challenge, but this has to also take into account the fact that a rich amount of older literature from some countries, when CL research was dominant, may be holding back their ratio, but that it does not mean that currently, these countries are just as engaged as others in CC/GW/CE research.
Figure 6 provides a different view of the country/region publication records. By plotting the number of CC/GW/CE publications for each country/region versus the number of CL publications in the same country/region, it is possible to see a focusing effect about the 1:1 dashed line. Countries that have published more, have more diverse body of literature and tend towards the 1:1 line (the USA is the highest point). Countries that have published less are more likely to be more engaged in recent research and thus have more CC/GW/CE articles than CL articles. Notable outliers with more than 10 CL publications (i.e., farthest from the 1:1 line and with a robust body of literature) are Fiji (with the ratio of 2.92) and Monaco (with the ratio of 4.82), both above the line.
4.2.1. Country collaboration
Figure S-6 shows the countries of collaboration of the first authors of the five most cited articles in the CC/GW/CE and CL records, based on the affiliations listed in these authors' articles. For the authors from the CC/GW/CE record, apart from the countries of affiliation of the authors (the USA, England and Austria), the most frequent countries of collaboration have been Germany, the USA, Australia, France and Spain. For the CL record, apart from the country of affiliation of the authors (all from the USA), the most frequent countries of collaboration have been England, Germany, Australia, Canada, China, and Japan. Notably, there is more variety in collaboration in the articles from the CL record. For example, Robert J. Hijmans has collaborated with Peru and the Philippines, which have serious problems with droughts and floods ( Elith et al., 2006 ; Fick and Hijmans, 2017 ). Perhaps unsurprisingly, these highly cited authors collaborate most with anglophone and European countries far more than with others. With climate changing affecting every country on Earth, and disproportionally threatening populations in smaller and less wealthy countries, it would be great to see in the near future deeper engagement of lead researchers directly with the scientific communities in those countries. Among the top 500 articles in the CC/GW/CE record some of these engagements are happing, as seen in Figure S-8 in the Supplementary Materials. Moreover, according to Figures S-5, S-7 and S-8 in the Supplementary Materials, the countries' scientific production and collaboration for the CC/GW/CE record are significantly more diverse than that of the CL record. As mentioned earlier, small island states are at most risk of the catastrophic effects of climate change, particularly rising sea levels ( Vitousek et al., 2017 ; Horton et al., 2014 ; Nunn, 2009 ; King and Harrington, 2018 ; Widlansky et al., 2015 ), which is reflected in the countries’ scientific production and collaboration maps for the CC/GW/CE record (Figures S-5 and S-8).
4.3. Source of publication
Table 3 , Figure 7 , present the data analysis for the source (i.e., journals indexed in WoS) of publication for a part of the records, ranging from 1910 to 2020. The sources analyzed are the top 20 venues of publications from each record in terms of the number of publications in each source. The top 20 were chosen to make the analysis manageable from a reporting and graphing perspective. The top 20 of the CC/GW/CE record represents 18.20% of all articles in this record, and the top 20 of the CL record represents a very similar 18.05% of that record. It is deemed that observations and trends made from the top 20 will be valid as a proxy for the trends of the full record.
Table 3
Top 20 sources of articles from the two records (CC/GW/CE and CL). The number of articles in each source, the percentage of the total number of articles in the full record, and the publication ratios ((CC/GW/CE)/(CL)). Bolded entries are the top 20 of each record, and bolded values reflect the entries that are top 20 on both records (i.e., “match”).
CC/GW/CE Sources ↓ | Articles | % of 245,391 | CL Sources ↓ | Articles | % of 228,280 | Ratio ((CC/GW/CE)/(CL)) |
---|---|---|---|---|---|---|
Atmospheric Chemistry and Physics | 761 | 0.310 | 2,420 | 1.060 | 0.31 | |
Atmospheric Environment | 638 | 0.260 | 1,001 | 0.438 | 0.64 | |
C | 3,458 | 1.409 | Climatic Change | 811 | 0.355 | 4.26 |
Earth and Planetary Science Letters | 707 | 0.288 | 1,053 | 0.461 | 0.67 | |
1,545 | 0.630 | Energy Policy | 623 | 0.273 | 2.48 | |
1,901 | 0.775 | Environmental Research Letters | 839 | 0.368 | 2.27 | |
Forest Ecology and Management | 1,416 | 0.577 | 921 | 0.403 | 1.54 | |
3,364 | 1.371 | Global Change Biology | 625 | 0.274 | 5.38 | |
2,323 | 0.947 | Journal of Cleaner Production | 487 | 0.213 | 4.77 | |
1,625 | 0.662 | Proceedings of the National Academy of Sciences of the United States of America (PNAS) | 849 | 0.372 | 1.91 | |
Remote Sensing | 1,220 | 0.497 | 1,189 | 0.521 | 1.03 | |
Theoretical and Applied Climatology | 1,207 | 0.492 | 1,412 | 0.619 | 0.85 | |
1,867 | 0.761 | Water | 704 | 0.308 | 2.65 | |
Water Resources Research | 923 | 0.376 | 1,001 | 0.438 | 0.92 |

Publication ratio ((CC/GW/CE)/(CL)) for 27 journals, categorized as ‘match’ or ‘non-match’ based on appearance or not in both top 20 lists, ordered from highest to lowest ratio (one curve contains 13 entries and the other 14).
Table 3 presents the number of articles from each record that appear in these journals. A total of 27 journals appear in Table 3 , organized in alphabetic order since there is a partial overlap of the top 20 from each record; in fact, there are 13 journals that are common to both top 20's (these are referred to as ‘match’ journals), and 14 journals in each top 20 that is not in the other top 20 (these are referred to as ‘non-match’ journals). The top journal in the CC/GW/CE record is PLOS ONE, with 3,602 articles representing 1.468% of the full record. The top journal in the CL record is the Journal of Climate, with 5,279 articles representing 2.284% of the full record. Both of these journals are in both top 20 lists, with PLOS ONE having a stronger record of CC/GW/CE articles as given by a ratio (as previously calculated to compare records) of 2.20, while the Journal of Climate has a stronger record of general CL research, with a 0.42 ratio. The ‘match’ journal with the highest ratio (2.36) is Science of the Total Environment, while the Journal of Geophysical Research Atmospheres has the lowest ratio of 0.34. The range of ratios is larger for ‘non-match’ journals. Here the highest ratio is 2.83 for the journal of Sustainability, and the smallest ratio is 0.31 for the journal of Atmospheric Chemistry and Physics. These ratios agree with the perception that can be taken about each of these journals. Journals like PLOS One, Science of the Total Environment and Global Change Biology appeal to more applied areas of research, including the applied sciences and engineering, and have wider aims and scopes, while journals such as the Journal of Climate, Journal of Geophysical Research Atmosphere and Atmospheric Chemistry and Physics appeal to more fundamental and specialized research.
Figure 7 helps to highlight the different scopes of the 27 journals listed in Table 3 . Here, the ratios for each journal are plotted and categorized according to ‘match’ or ‘non-match’ journals. The spider plot shows data arranged from largest to smallest ratio for each category (i.e., each line). Had there been no significant difference in the distribution of the articles across the various journals, both of these lines would be very close to the value of 1. It is clear that the ‘non-match’ line deviates the most from 1, with nearly every value much higher or much lower than 1. Even the ‘match’ line deviates significantly from 1. The ratio value closest to 1 from both categories is that of the journal Remote Sensing (0.94). This is not surprising as remote sensing is a climate monitoring technique that can be used both for general climate research as well as to track changes in the climate due to anthropogenic effects ( Kuenzer et al., 2011 , Levizzani and Cattani, 2019 ; Milesi and Churkina, 2020 ). What can be concluded from this analysis is that journals and authors are able to distinguish the research topics sufficiently to steer more CC/GW/CE research to certain journals and more general CL research to other journals. This helps to confirm that these two topics are distinct in practice.
4.4. Organization of publication
Table 4 , Figure 8 , present the data analysis for organization (i.e., universities, research institutes, and other research-intensive organizations, associated with the corresponding author's primary affiliation) of publication, for a part of the records, ranging from 1910 to 2020. As with sources, the organizations analyzed are the top 20 from each record, and an identical data analysis procedure was used here. The top 20 of the CC/GW/CE record represents 36.72% of all articles in this record, and the top 20 of the CL record represents a slightly higher 38.53% of that record. A total of 26 organizations appear in Table 4 , signifying that there are 14 common organizations within the top 20 (i.e., ‘match’ organizations), and 12 ‘non-match’ organizations. Here, the top three organizations are the same on both records, with the Chinese Academy of Sciences (China) being slightly more engaged in CC/GW/CE research than the the University of California System (USA) and the Centre National de la Recherche Scientifique (France); the ratios of these three organizations are very close to 1 (1.22, 1.05, and 1.02, respectively). In fact, the ratios of these organizations are much closer to 1, on average, than those of the journals. The highest ratio among the 26 organizations is 1.87 for the United States Forest Service, and the lowest ratio is 0.43 for the National Aeronautics Space Administration. This suggests that academic organizations may have more varied research, and hence ratios closer to 1, while governmental organizations may be more focused on a particular line of research, and thus rations more different than 1. Although such a conclusion would require analysis of a large set of organizations, and is complicated by some countries having organizations that have a dual academic and institutional role.
Table 4
Top 20 organizations of articles from the two records (CC/GW/CE and CL). The number of articles from each organization, the percentage of total number of articles in the full record, and the publication ratios ((CC/GW/CE)/(CL)). Bolded entries are the top 20 of each record, and bolded values reflect the entries that are top 20 on both records (i.e., “match”).
CC/GW/CE Organizations ↓ | Articles | % of 245,391 | CL Organizations ↓ | Articles | % of 228,280 | Ratio ((CC/GW/CE)/(CL)) |
---|---|---|---|---|---|---|
Columbia University | 2,358 | 0.961 | 2,945 | 1.290 | 0.80 | |
3,348 | 1.364 | Commonwealth Scientific Industrial Research Organisation (CSIRO) | 2,500 | 1.095 | 1.34 | |
2,872 | 1.170 | Institut national de recherche pour l'agriculture, l'alimentation et l'environnement (INRAE) | 1,946 | 0.852 | 1.48 | |
Max Planck Society | 1,969 | 0.802 | 2,723 | 1.193 | 0.72 | |
National Aeronautics Space Administration (NASA) | 2,479 | 1.010 | 5,713 | 2.502 | 0.43 | |
National Center for Atmospheric Research (NCAR) | 1,952 | 0.795 | 3,643 | 1.596 | 0.54 | |
2,533 | 1.032 | United States Forest Service | 1,354 | 0.593 | 1.87 | |
3,670 | 1.496 | United States Geological Survey | 2,462 | 1.078 | 1.49 | |
University of Colorado Boulder | 2,343 | 0.955 | 3,339 | 1.462 | 0.70 | |
3,100 | 1.263 | University of London | 2,307 | 1.010 | 1.34 | |
University System of Maryland | 1,850 | 0.754 | 2,620 | 1.147 | 0.71 | |
2,634 | 1.073 | Wageningen University Research | 1,482 | 0.649 | 1.78 |

Publication ratio ((CC/GW/CE)/(CL)) for 26 organizations, categorized as ‘match’ or ‘non-match’ based on appearance or not in both top 20 lists, ordered from highest to lowest ratio (one curve contains 14 entries and the other 12).
Figure 8 , in contrast to Figure 7 , makes it clear that organizational information is not enough to place research as belonging to one record or another. Both the ‘match’ and ‘non-match’ lines deviated by small extents away from the ratio of 1 level, with the ‘non-match’ line deviating more, as would be expected. For comparison with the earlier case, one of the ratio values closest to 1 from both categories is that of the University of California System (1.05). Notable about this organization is that it consists of nine campuses offering comprehensive education, with varying levels of research excellence (e.g., excellent (Berkeley), very good (Davis), less highly ranked (Riverside), emerging (Merced) ( Gibson et al., 2014 ). This can explain the diversity of research output, covering both CC/GW/CE and CL topics. Of course, this diversity of topic is an average since 1900, and it is possible that in recent years the research in many organizations has shifted towards the climate challenge, as the year and country trends presented earlier suggest.
5. Discussion
Here, we can revisit the three hypotheses and reach conclusions about their claims. With regards to hypothesis 1, it was possible to distinguish the scientific literature linked to CC/GW/CE research from that pertaining to CL research using the two search strings tested. This was verified by reviewing the scope of a number of highly cited papers in both records and is further supported by the trends seen with regards to years of publication, country/region of publication, and source of publication. That is, in these three categories, the publication records showed significant numerical and graphical differences, and these could at times be explained rationally, with basis on data interpretation. Evidence was found that the two publication records contained some misinterpreted publications and outliers, but it is deemed that the consistency of trends observed signify that these issues are minor and acceptable given the simplicity of the publication record assemblage method. A third search string, related to the topic of greenhouse gases, was also tested, but its data did not become part of this study's analysis. The reason for this is that such search string finds many articles that discuss the emissions of greenhouse gases (e.g., from flue gas stacks ( Su et al., 2009 ) or from livestock farming ( Herrero et al., 2013 )) and technologies to control or mitigate these emissions (e.g., via carbon sequestration ( Santos et al., 2013 ) or green energy ( Panepinto et al., 2013 )). As such, these articles fall outside the scope of the two topical records of interest here. This is further evidence that the two search strings used are effective in reaching their intended goal.
With hypothesis 1 confirmed, it is also possible to confirm hypothesis 2. The publication year data clearly shows that the scientific literature has become enriched in CC/GW/CE works in relation to CL works. This is despite both of these records experiencing massive growth over the decades (from under 100 articles per year in the first half of the 20 th century to over 10,000 articles per year in recent years). It is clear that the more pressing the climate challenge becomes, and its effects actually witnessed ( Mahé et al., 2013 ), the more research is being undertaken to forecast the avoidable or unavoidable impacts ( Ito et al., 2020 ; Döll et al., 2020 ). It is difficult to foresee for how much longer the publication ratio devised in this study will continue to rise, globally or country-by-country, as climate research will become increasingly important in the framework of a sustainable society, so it will be worth revisiting this in a decade or more. Perhaps by then another keyword could be added to complement CC/GW/CE. As of August 7 th 2021, 85.82% of this record is retrieved using only CC and excluding (via the NOT operator) the other two search terms (("climat∗ chang∗" NOT ("global warming∗" OR "climat∗ emergenc∗"))); this compares to only 8.32% of the record that only contains GW (("global warming∗" NOT ("climat∗ chang∗" OR "climat∗ emergenc∗"))), and a mere 0.0248% of the record that only contains CE (("climat∗ emergenc∗" NOT ("global warming∗" OR "climat∗ chang∗"))). A simple search for CE yields 61 articles, 53 of which published since 2019, and the oldest from 2011 ( McMichael, 2011 ) being the most cited to date. This shows that this popular term (in the greater public sphere) is not yet commonly used scientifically; will it eventually be?
Hypothesis 3 was partly confirmed. The data and its interpretation show that the two publications records have distinct differences in terms of size (i.e., the number of publications) when it comes to the originating country/region and venue (journal) of publication. Yet, the two records are nearly indistinguishable when the criteria used are the organizations responsible for producing the work. As was explained, research organizations have broad research interests, and it is understandable that the same departments and research groups that perform CC/GW/CE research also tend to perform CL research. Of course, this would not be the case at the researcher level since expertise for these two topics of research is sufficiently different. WoS allows data analysis at the researcher (i.e., corresponding author level). However, in addition to the number of entries being very large (there are over 100,000 corresponding authors listed in the most recent CC/GW/CE and CL records), there is ambiguity with common author names (i.e., same last name and the same first letter of the first name), making any possible analysis less accurate. Such analysis would thus require close scrutiny at the article level.
6. Conclusions
This article presented and discussed the scientific publication record from 1910 to 2020 on two topics: "climate" and "climate change/global warming/climate emergency". The goal is to comparatively visualize how these two distinct publication records have evolved over time, from different classification perspectives, using publication ratios as the key indicator, which were presented as three hypotheses. To test our hypotheses, we defined publication ratios as the number of publications in a category in one record over that in another record, which allowed us to distinguish and contrast CC/GW/CE versus general CL research. The hypotheses can also be expressed as the following questions: (i) what are the dynamics of the conceptual structure of CC/GW/CE versus general CL research; (ii) when has the scientific record in CC/GW/CE versus general climate (CL) research become more enriched; (iii) which countries have made the climate challenge the dominant topic, and are there any links between countries and the dominant scientific topic? The following are the answers to these questions, which present the study's conclusions:
- - The journal name and scope had a direct relationship with the number and ratio of publications in these two records; for example, journals like PLOS One, Science of the Total Environment, and Global Change Biology appealed to more applied areas of research, including the applied sciences and engineering, and have wider aims and scopes, while journals such as the Journal of Climate, Journal of Geophysical Research Atmosphere, and Journal of the Atmospheric Sciences appealed to more fundamental and specialized research.
- - Governmental organizations focused more on a specific line of research (publication ratios farther from a value of 1), whereas academic organizations' research areas were broader and covered a wide range of topics (publication ratios closer to 1).
- - It was discovered that research output related to the Earth's current changing climate surpassed that of general climate research in 2010, and the publication ratio (CC/GW/CE)/(CL) has been increasing over the last decade.
- - Among other countries, island states such as the Philippines, Fiji, Bahamas, Palau, Micronesia, and Kiribati had the highest ratios, highlighting the fact that small island states are most vulnerable to the catastrophic effects of climate change, particularly rising sea levels.
- - Ideas for future bibliometric studies that employ our hypothesis-driven approach and the use of publication ratios as the key trends indicator include: (i) inspecting more closely how non-scientific publications, such as those indexed by databases such as SSCI and A&HCI, have been evolving in covering the topics of climate change, global warming and the climate emergency; (ii) comparing the scientific literature that studies the causes and effects of climate change to the scientific literature that develops ways of mitigating or adapting to climate change or being resilient to it; (iii) identifying important topical gaps in the literature review record (e.g., well-cited articles or articles published in high impact journals that have not been covered in literature reviews); among other possibilities.
Declarations
Author contribution statement.
Rafael M. Santos: Conceived and designed the experiments; Analyzed and interpreted the data; Wrote the paper.
Reza Bakhshoodeh: Performed the experiments; Analyzed and interpreted the data; Contributed reagents, materials, analysis tools or data; Wrote the paper.
Funding statement
This research did not receive any specific grant from funding agencies in the public, commercial, or not-for-profit sectors.
Data availability statement
Declaration of interests statement.
The authors declare no conflict of interest.
Additional information
No additional information is available for this paper.
Acknowledgements
The authors acknowledge the University of Guelph Library and the University of Western Australia Library for the Web of Science access provided, which made this study possible.
Appendix A. Supplementary data
The following is the supplementary data related to this article:
- Aleixandre-Benavent R., Aleixandre-Tudó J.L., Castelló-Cogollos L., Aleixandre J.L. Trends in scientific research on climate change in agriculture and forestry subject areas (2005–2014) J. Clean. Prod. 2017; 147 :406–418. [ Google Scholar ]
- Allen C.D., Macalady A.K., Chenchouni H., Bachelet D., Mcdowell N., Vennetier M., Kitzberger T., Rigling A., Breshears D.D., Hogg E.T. A global overview of drought and heat-induced tree mortality reveals emerging climate change risks for forests. For. Ecol. Manag. 2010; 259 :660–684. [ Google Scholar ]
- Arana Barbier P.J. Situation of trust in grounded theory versus structural equation modeling: a comparative bibliometric study for management. Invest. Bibl. 2020; 34 :37–53. [ Google Scholar ]
- Aria M., Cuccurullo C. bibliometrix: an R-tool for comprehensive science mapping analysis. J. Inf. 2017; 11 (4):959–975. [ Google Scholar ]
- Baek S., Yoon D.Y., Lim K.J., Hong J.H., Moon J.Y., Seo Y.L., Yun E.J. Top-cited articles versus top Altmetric articles in nuclear medicine: a comparative bibliometric analysis. Acta Radiol. 2020; 61 :1343–1349. [ PubMed ] [ Google Scholar ]
- Benton G.S. Carbon dioxide and its role in climate change. Proc. Natl. Acad. Sci. U.S.A. 1970; 67 :898. [ PMC free article ] [ PubMed ] [ Google Scholar ]
- Biesmeijer J.C., Roberts S.P.M., Reemer M., Ohlemuller R., Edwards M., Peeters T., Schaffers A.P., Potts S.G., Kleukers R., Thomas C.D., Settele J., Kunin W.E. Parallel declines in pollinators and insect-pollinated plants in Britain and The Netherlands. Science. 2006; 313 :351–354. [ PubMed ] [ Google Scholar ]
- Bornmann L., Marx W. Distributions instead of single numbers: percentiles and beam plots for the assessment of single researchers. J. Assoc. Inf. Sci. Technol. 2014; 65 :206–208. [ Google Scholar ]
- Breshears D.D., Cobb N.S., Rich P.M., Price K.P., Allen C.D., Balice R.G., Romme W.H., Kastens J.H., Floyd M.L., Belnap J., Anderson J.J., Myers O.B., Meyer C.W. Regional vegetation die-off in response to global-change-type drought. Proc. Natl. Acad. Sci. U.S.A. 2005; 102 :15144–15148. [ PMC free article ] [ PubMed ] [ Google Scholar ]
- Bustreo C., Giuliani U., Maggio D., Zollino G. How fusion power can contribute to a fully decarbonized European power mix after 2050. Fusion Eng. Des. 2019; 146 :2189–2193. [ Google Scholar ]
- Callendar G.S. The artificial production of carbon dioxide and its influence on temperature. Q. J. R. Meteorol. Soc. 1938; 64 :223–240. [ Google Scholar ]
- Chen I.C., Hill J.K., Ohlemuller R., Roy D.B., Thomas C.D. Rapid range shifts of species associated with high levels of climate warming. Science. 2011; 333 :1024–1026. [ PubMed ] [ Google Scholar ]
- Demiroglu O.C., Hall C.M. Geobibliography and bibliometric networks of polar tourism and climate change research. Atmosphere. 2020; 11 :498. [ Google Scholar ]
- Döll P., Trautmann T., Göllner M., Schmied H.M. A global-scale analysis of water storage dynamics of inland wetlands: quantifying the impacts of human water use and man-made reservoirs as well as the unavoidable and avoidable impacts of climate change. Ecohydrology. 2020; 13 [ Google Scholar ]
- Elith J., Graham C.H., Anderson R.P., Dudik M., Ferrier S., Guisan A., Hijmans R.J., Huettmann F., Leathwick J.R., Lehmann A., Li J., Lohmann L.G., Loiselle B.A., Manion G., Moritz C., Nakamura M., Nakazawa Y., Overton J.M., Peterson A.T., Phillips S.J., Richardson K., Scachetti-Pereira R., Schapire R.E., Soberon J., Williams S., Wisz M.S., Zimmermann N.E. Novel methods improve prediction of species' distributions from occurrence data. Ecography. 2006; 29 :129–151. [ Google Scholar ]
- Fang Y., Yin J., Wu B. Climate change and tourism: a scientometric analysis using CiteSpace. J. Sustain. Tourism. 2018; 26 :108–126. [ Google Scholar ]
- Fawzy S., Osman A.I., Doran J., Rooney D.W. Strategies for mitigation of climate change: a review. Environ. Chem. Lett. 2020; 18 :2069–2094. [ Google Scholar ]
- Feinberg, J. 2020. Jonathan Feinberg. http://mrfeinberg.com/ .
- Fick S.E., Hijmans R.J. WorldClim 2: new 1-km spatial resolution climate surfaces for global land areas. Int. J. Climatol. 2017; 37 :4302–4315. [ Google Scholar ]
- Frisken W. Extended industrial revolution and climate change. EOS Trans. Am. Geophys. Union. 1971; 52 :500–508. [ Google Scholar ]
- Fu H.-Z., Waltman L. 2021. A Large-Scale Bibliometric Analysis of Global Climate Change Research between 2001 and 2018. arXiv preprint arXiv:2107.08214. [ Google Scholar ]
- Gibson J., Anderson D.L., Tressler J. Which journal rankings best explain academic salaries? Evidence from the University of California. Econ. Inq. 2014; 52 :1322–1340. [ Google Scholar ]
- Goglio P., Williams A.G., Balta-Ozkan N., Harris N.R., Williamson P., Huisingh D., Zhang Z., Tavoni M. Advances and challenges of life cycle assessment (LCA) of greenhouse gas removal technologies to fight climate changes. J. Clean. Prod. 2020; 244 :118896. [ Google Scholar ]
- Haunschild R., Bornmann L., Marx W. Climate change research in view of bibliometrics. PLoS One. 2016; 11 (7) [ PMC free article ] [ PubMed ] [ Google Scholar ]
- Herrero M., Havlík P., Valin H., Notenbaert A., Rufino M.C., Thornton P.K., Blümmel M., Weiss F., Grace D., Obersteiner M. Biomass use, production, feed efficiencies, and greenhouse gas emissions from global livestock systems. Proc. Natl. Acad. Sci. Unit. States Am. 2013; 110 :20888–20893. [ PMC free article ] [ PubMed ] [ Google Scholar ]
- Hijmans R.J., Cameron S.E., Parra J.L., Jones P.G., Jarvis A. Very high resolution interpolated climate surfaces for global land areas. Int. J. Climatol. 2005; 25 :1965–1978. [ Google Scholar ]
- Hisano M., Searle E.B., Chen H.Y. Biodiversity as a solution to mitigate climate change impacts on the functioning of forest ecosystems. Biol. Rev. 2018; 93 :439–456. [ PubMed ] [ Google Scholar ]
- Horton B.P., Rahmstorf S., Engelhart S.E., Kemp A.C. Expert assessment of sea-level rise by AD 2100 and AD 2300. Quat. Sci. Rev. 2014; 84 :1–6. [ Google Scholar ]
- Ihaka R. Statistics Department, The University of Auckland; Auckland, New Zealand: 1998. R : Past and Future History (Technical Report). Interface '98. [ Google Scholar ]
- IPCC . In: Climate Change 2014: Synthesis Report. Contribution of Working Groups I, II and III to the Fifth Assessment Report of the Intergovernmental Panel on Climate Change [Core Writing Team. Pachauri R.K., Meyer L.A., editors. IPCC; Geneva, Switzerland: 2014. p. 151. [ Google Scholar ]
- IPCC . In: In: Global Warming of 1.5°C. An IPCC Special Report on the Impacts of Global Warming of 1.5°C above Pre-industrial Levels and Related Global Greenhouse Gas Emission Pathways, in the Context of Strengthening the Global Response to the Threat of Climate Change, Sustainable Development, and Efforts to Eradicate Poverty. Matthews J.B.R., editor. 2018. Annex I: glossary. [Masson-Delmotte, V., P. Zhai, H.-O. Pörtner, D. Roberts, J. Skea, P.R. Shukla, A. Pirani, W. Moufouma-Okia, C. Péan, R. Pidcock, S. Connors, J.B.R. Matthews, Y. Chen, X. Zhou, M.I. Gomis, E. Lonnoy, T. Maycock, M. Tignor, and T. Waterfield (eds.)]. In Press. [ Google Scholar ]
- Ito A., Reyer C.P., Gädeke A., Ciais P., Chang J., Chen M., François L., Forrest M., Hickler T., Ostberg S. Pronounced and unavoidable impacts of low-end global warming on northern high-latitude land ecosystems. Environ. Res. Lett. 2020; 15 [ Google Scholar ]
- Janmaijaya M., Shukla A.K., Abraham A., Muhuri P.K. Scientometric study of neurocomputing publications (1992–2018): An aerial overview of intrinsic structure. Publications. 2018; 6 [ Google Scholar ]
- Janssen M.A., Schoon M.L., Ke W., Börner K. Scholarly networks on resilience, vulnerability and adaptation within the human dimensions of global environmental change. Global Environ. Change. 2006; 16 :240–252. [ Google Scholar ]
- Kalnay E., Kanamitsu M., Kistler R., Collins W., Deaven D., Gandin L., Iredell M., Saha S., White G., Woollen J., Zhu Y., Chelliah M., Ebisuzaki W., Higgins W., Janowiak J., Mo K.C., Ropelewski C., Wang J., Leetmaa A., Reynolds R., Jenne R., Joseph D. The NCEP/NCAR 40-year reanalysis project. Bull. Am. Meteorol. Soc. 1996; 77 :437–471. [ Google Scholar ]
- Khalidy R., Santos R.M. The fate of atmospheric carbon sequestrated through weathering in mine tailings. Miner. Eng. 2021; 163 :106767. [ Google Scholar ]
- King A.D., Harrington L.J. The inequality of climate change from 1.5 to 2 °C of global warming. Geophys. Res. Lett. 2018; 45 :5030–5033. [ Google Scholar ]
- Kistler R., Kalnay E., Collins W., Saha S., White G., Woollen J., Chelliah M., Ebisuzaki W., Kanamitsu M., Kousky V., Van Den Dool H., Jenne R., Fiorino M. The NCEP-NCAR 50-year reanalysis: monthly means CD-ROM and documentation. Bull. Am. Meteorol. Soc. 2001; 82 :247–267. [ Google Scholar ]
- Kopec R.J. Global climate change and the impact of a maximum sea level on coastal settlement. J. Geogr. 1971; 70 :541–550. [ Google Scholar ]
- Kottek M., Grieser J., Beck C., Rudolf B., Rubel F. World map of the Köppen-Geiger climate classification updated. Meteorol. Z. 2006; 15 (3):259–263. [ Google Scholar ]
- Kuenzer C., Bluemel A., Gebhardt S., Quoc T.V., Dech S. Remote sensing of mangrove ecosystem: a review. Rem. Sens. 2011; 3 :878–928. [ Google Scholar ]
- Kyte R. Gaidar Forum Moscow, Russian Federation; 2014. Climate Change Is a Challenge for Sustainable Development. https://www.worldbank.org/en/news/speech/2014/01/15/climate-change-is-challenge-for-sustainable-development URL. [ Google Scholar ]
- Levizzani V., Cattani E. Satellite remote sensing of precipitation and the terrestrial water cycle in a changing climate. Rem. Sens. 2019; 11 :2301. [ Google Scholar ]
- Lezaun J. Hugging the shore: tackling marine carbon dioxide removal as a local governance problem. Front. Climate. 2021; 3 :684063. [ Google Scholar ]
- Li F., Zhou H., Huang D.-S., Guan P. Global research output and theme trends on climate change and infectious diseases: a restrospective bibliometric and Co-word biclustering investigation of papers indexed in PubMed (1999–2018) Int. J. Environ. Res. Publ. Health. 2020; 17 :5228. [ PMC free article ] [ PubMed ] [ Google Scholar ]
- Li J., Wang M.-H., Ho Y.-S. Trends in research on global climate change: a Science Citation Index Expanded-based analysis. Global Planet. Change. 2011; 77 :13–20. [ Google Scholar ]
- Lockley A., Mi Z., Coffman D.M. Geoengineering and the blockchain: coordinating carbon dioxide removal and solar radiation management to tackle future emissions. Front. Eng. Manag. 2019; 6 :38–51. [ Google Scholar ]
- Lockyer W.J. Does the Indian climate change? Nature. 1910; 84 :178. [ Google Scholar ]
- Macchi Silva V.V., Ribeiro J.L.D., Alvarez G.R., Caregnato S.E. Competence-based management research in the web of science and scopus databases: scientific production, collaboration, and impact. Publications. 2019; 7 [ Google Scholar ]
- Mahé G., Lienou G., Descroix L., Bamba F., Paturel J.-E., Laraque A., Meddi M., Habaieb H., Adeaga O., Dieulin C. The rivers of Africa: witness of climate change and human impact on the environment. Hydrol. Process. 2013; 27 :2105–2114. [ Google Scholar ]
- Martens P., Mcevoy D., Chang C.T. Sustainability Science. Springer; 2016. Climate change: responding to a major challenge for sustainable development. [ Google Scholar ]
- Marx W., Haunschild R., Bornmann L. Global warming and tea production—the bibliometric view on a newly emerging research topic. Climate. 2017; 5 :46. [ Google Scholar ]
- Mcdowell N., Pockman W.T., Allen C.D., Breshears D.D., Cobb N., Kolb T., Plaut J., Sperry J., West A., Williams D.G., Yepez E.A. Mechanisms of plant survival and mortality during drought: why do some plants survive while others succumb to drought? New Phytol. 2008; 178 :719–739. [ PubMed ] [ Google Scholar ]
- Mcmichael P. Food system sustainability: questions of environmental governance in the new world (dis)order. Global Environ. Change. 2011; 21 :804–812. [ Google Scholar ]
- Meehl G.A., Covey C., Delworth T., Latif M., Mcavaney B., Mitchell J.F.B., Stouffer R.J., Taylor K.E. The WCRP CMIP3 multimodel dataset - a new era in climate change research. Bull. Am. Meteorol. Soc. 2007; 88 :1383–1394. [ Google Scholar ]
- Mesinger F., Dimego G., Kalnay E., Mitchell K., Shafran P.C., Ebisuzaki W., Jovic D., Woollen J., Rogers E., Berbery E.H., Ek M.B., Fan Y., Grumbine R., Higgins W., Li H., Lin Y., Manikin G., Parrish D., Shi W. North American regional reanalysis. Bull. Am. Meteorol. Soc. 2006; 87 :343–360. [ Google Scholar ]
- Milán-García J., Caparrós-Martínez J.L., Rueda-López N., De Pablo Valenciano J. Climate change-induced migration: a bibliometric review. Glob. Health. 2021; 17 :1–10. [ PMC free article ] [ PubMed ] [ Google Scholar ]
- Milesi C., Churkina G. Measuring and monitoring urban impacts on climate change from space. Rem. Sens. 2020; 12 :3494. [ Google Scholar ]
- Munasinghe M. Addressing the sustainable development and climate change challenges together: applying the sustainomics framework. Procedia Social Behavl. Sci. 2010; 2 :6634–6640. [ Google Scholar ]
- Nunn P.D. Responding to the challenges of climate change in the Pacific Islands: management and technological imperatives. Clim. Res. 2009; 40 :211–231. [ Google Scholar ]
- Oliveira C.Y.B., Oliveira C.D.L., Müller M.N., Santos E.P., Dantas D.M., Gálvez A.O. A scientometric overview of global dinoflagellate research. Publications. 2020; 8 :50. [ Google Scholar ]
- Osman A.I., Hefny M., Maksoud M.A., Elgarahy A.M., Rooney D.W. Recent advances in carbon capture storage and utilisation technologies: a review. Environ. Chem. Lett. 2020; 19 :797–849. [ Google Scholar ]
- Ouhamdouch S., Bahir M., Ouazar D., Carreira P.M., Zouari K. Evaluation of climate change impact on groundwater from semi-arid environment (Essaouira Basin, Morocco) using integrated approaches. Environ. Earth Sci. 2019; 78 :1–14. [ Google Scholar ]
- Panepinto D., Genon G., Brizio E., Russolillo D. Production of green energy from co-digestion: perspectives for the Province of Cuneo, energetic balance and environmental sustainability. Clean Technol. Environ. Policy. 2013; 15 :1055–1062. [ Google Scholar ]
- Parmesan C. Ecological and evolutionary responses to recent climate change. Annu. Rev. Ecol. Evol. Systemat. 2006; 37 :637–669. [ Google Scholar ]
- Parmesan C., Yohe G. A globally coherent fingerprint of climate change impacts across natural systems. Nature. 2003; 421 :37–42. [ PubMed ] [ Google Scholar ]
- Phillips S.J., Anderson R.P., Schapire R.E. Maximum entropy modeling of species geographic distributions. Ecol. Model. 2006; 190 :231–259. [ Google Scholar ]
- Phillips S.J., Dudik M. Modeling of species distributions with Maxent: new extensions and a comprehensive evaluation. Ecography. 2008; 31 :161–175. [ Google Scholar ]
- Princiotta F.T., Loughlin D.H. Global climate change: the quantifiable sustainability challenge. J. Air Waste Manag. Assoc. 2014; 64 :979–994. [ PubMed ] [ Google Scholar ]
- R core team . R Foundation for Statistical Computing; Vienna, Austria: 2019. R: A Language and Environment for Statistical Computing. https://www.R-project.org/ URL. [ Google Scholar ]
- Rayner N.A., Parker D.E., Horton E.B., Folland C.K., Alexander L.V., Rowell D.P., Kent E.C., Kaplan A. Global analyses of sea surface temperature, sea ice, and night marine air temperature since the late nineteenth century. J. Geophys. Res.: Atmosphere. 2003; 108 :37. [ Google Scholar ]
- Reynolds R.W., Rayner N.A., Smith T.M., Stokes D.C., Wang W.Q. An improved in situ and satellite SST analysis for climate. J. Clim. 2002; 15 :1609–1625. [ Google Scholar ]
- Ricke K., Millar R., Macmartin D.G. Constraints on global temperature target overshoot. Sci. Rep. 2017; 7 :1–7. [ PMC free article ] [ PubMed ] [ Google Scholar ]
- Rubel F., Kottek M. Observed and projected climate shifts 1901-2100 depicted by world maps of the Koppen-Geiger climate classification. Meteorol. Z. 2010; 19 :135–141. [ Google Scholar ]
- Rubel F., Kottek M. The thermal zones of the earth" by Wladimir Koppen (1884) Meteorol. Z. 2011; 20 :361–365. [ Google Scholar ]
- Salmerón-Manzano E., Manzano-Agugliaro F. Worldwide scientific production indexed by scopus on labour relations. Publications. 2017; 5 [ Google Scholar ]
- Santos R.M., Verbeeck W., Knops P., Rijnsburger K., Pontikes Y., Van Gerven T. Integrated mineral carbonation reactor technology for sustainable carbon dioxide sequestration: ‘CO 2 Energy Reactor. Energy Procedia. 2013; 37 :5884–5891. [ Google Scholar ]
- Sobreira C., Klu J.K., Cole C., Nic Daéid N., Ménard H. Reviewing research trends—a scientometric approach using gunshot residue (GSR) literature as an example. Publications. 2020; 8 :7. [ Google Scholar ]
- Steyvers M., Tenenbaum J.B. The large-scale structure of semantic networks: statistical analyses and a model of semantic growth. Cognit. Sci. 2005; 29 :41–78. [ PubMed ] [ Google Scholar ]
- Su F., Lu C., Cnen W., Bai H., Hwang J.F. Capture of CO 2 from flue gas via multiwalled carbon nanotubes. Sci. Total Environ. 2009; 407 :3017–3023. [ PubMed ] [ Google Scholar ]
- Tan X.-C., Zhu K.-W., Sun Y.-L., Zhao W.-Y., Chen F. Bibliometric research on the development of climate change in the BRI regions. Adv. Clim. Change Res. 2021; 12 (2):254–262. [ Google Scholar ]
- Taylor K.E. Summarizing multiple aspects of model performance in a single diagram. J. Geophys. Res.: Atmosphere. 2001; 106 :7183–7192. [ Google Scholar ]
- Taylor K.E., Stouffer R.J., Meehl G.A. An overview of CMIP5 and the experiment design. Bull. Am. Meteorol. Soc. 2012; 93 :485–498. [ Google Scholar ]
- Tenenbaum J.B., De Silva V., Langford J.C. A global geometric framework for nonlinear dimensionality reduction. Science. 2000; 290 :2319–2323. [ PubMed ] [ Google Scholar ]
- Tenenbaum J.B., Kemp C., Griffiths T.L., Goodman N.D. How to grow a mind: statistics, structure, and abstraction. Science. 2011; 331 :1279–1285. [ PubMed ] [ Google Scholar ]
- Thomas C.D., Cameron A., Green R.E., Bakkenes M., Beaumont L.J., Collingham Y.C., Erasmus B.F., De Siqueira M.F., Grainger A., Hannah L. Extinction risk from climate change. Nature. 2004; 427 :145–148. [ PubMed ] [ Google Scholar ]
- Uppala S.M., Kallberg P.W., Simmons A.J., Andrae U., Bechtold V.D., Fiorino M., Gibson J.K., Haseler J., Hernandez A., Kelly G.A., Li X., Onogi K., Saarinen S., Sokka N., Allan R.P., Andersson E., Arpe K., Balmaseda M.A., Beljaars A.C.M., Van de Berg L., Bidlot J., Bormann N., Caires S., Chevallier F., Dethof A., Dragosavac M., Fisher M., Fuentes M., Hagemann S., Holm E., Hoskins B.J., Isaksen L., Janssen P., Jenne R., Mcnally A.P., Mahfouf J.F., Morcrette J.J., Rayner N.A., Saunders R.W., Simon P., Sterl A., Trenberth K.E., Untch A., Vasiljevic D., Viterbo P., Woollen J. The ERA-40 re-analysis. Q. J. R. Meteorol. Soc. 2005; 131 :2961–3012. [ Google Scholar ]
- Vitousek S., Barnard P.L., Fletcher C.H., Frazer N., Erikson L., Storlazzi C.D. Doubling of coastal flooding frequency within decades due to sea-level rise. Sci. Rep. 2017; 7 :1–9. [ PMC free article ] [ PubMed ] [ Google Scholar ]
- Walther G.R., Post E., Convey P., Menzel A., Parmesan C., Beebee T.J.C., Fromentin J.M., Hoegh-Guldberg O., Bairlein F. Ecological responses to recent climate change. Nature. 2002; 416 :389–395. [ PubMed ] [ Google Scholar ]
- Wang B., Pan S.-Y., Ke R.-Y., Wang K., Wei Y.-M. An overview of climate change vulnerability: a bibliometric analysis based on Web of Science database. Nat. Hazards. 2014; 74 :1649–1666. [ Google Scholar ]
- Wang Y., Xie Y., Zhou R., Hu X., Li X. A comparative bibliometric analysis of Chinese and foreign articles in environmental footprint family (EFF) research. Environ. Sci. Pollut. Control Ser. 2021; 28 :26280–26293. [ PubMed ] [ Google Scholar ]
- Wei Y.-M., Mi Z.-F., Huang Z. Climate policy modeling: an online SCI-E and SSCI based literature review. Omega. 2015; 57 :70–84. [ Google Scholar ]
- Widlansky M.J., Timmermann A., Cai W. Future extreme sea level seesaws in the tropical Pacific. Sci. Adv. 2015; 1 (8) [ PMC free article ] [ PubMed ] [ Google Scholar ]
Thank you for visiting nature.com. You are using a browser version with limited support for CSS. To obtain the best experience, we recommend you use a more up to date browser (or turn off compatibility mode in Internet Explorer). In the meantime, to ensure continued support, we are displaying the site without styles and JavaScript.
- View all journals
- Explore content
- About the journal
- Publish with us
- Sign up for alerts
- Open access
- Published: 07 August 2024
Highest ocean heat in four centuries places Great Barrier Reef in danger
- Benjamin J. Henley ORCID: orcid.org/0000-0003-3940-1963 1 , 2 , 3 ,
- Helen V. McGregor ORCID: orcid.org/0000-0002-4031-2282 1 , 2 ,
- Andrew D. King ORCID: orcid.org/0000-0001-9006-5745 4 , 5 ,
- Ove Hoegh-Guldberg ORCID: orcid.org/0000-0001-7510-6713 6 ,
- Ariella K. Arzey 1 , 2 ,
- David J. Karoly 4 ,
- Janice M. Lough 7 ,
- Thomas M. DeCarlo ORCID: orcid.org/0000-0003-3269-1320 8 , 9 &
- Braddock K. Linsley ORCID: orcid.org/0000-0003-2085-0662 10
Nature volume 632 , pages 320–326 ( 2024 ) Cite this article
19k Accesses
1 Citations
3013 Altmetric
Metrics details
- Climate change
- Environmental impact
- Palaeoclimate
Mass coral bleaching on the Great Barrier Reef (GBR) in Australia between 2016 and 2024 was driven by high sea surface temperatures (SST) 1 . The likelihood of temperature-induced bleaching is a key determinant for the future threat status of the GBR 2 , but the long-term context of recent temperatures in the region is unclear. Here we show that the January–March Coral Sea heat extremes in 2024, 2017 and 2020 (in order of descending mean SST anomalies) were the warmest in 400 years, exceeding the 95th-percentile uncertainty limit of our reconstructed pre-1900 maximum. The 2016, 2004 and 2022 events were the next warmest, exceeding the 90th-percentile limit. Climate model analysis confirms that human influence on the climate system is responsible for the rapid warming in recent decades. This attribution, together with the recent ocean temperature extremes, post-1900 warming trend and observed mass coral bleaching, shows that the existential threat to the GBR ecosystem from anthropogenic climate change is now realized. Without urgent intervention, the iconic GBR is at risk of experiencing temperatures conducive to near-annual coral bleaching 3 , with negative consequences for biodiversity and ecosystems services. A continuation on the current trajectory would further threaten the ecological function 4 and outstanding universal value 5 of one of Earth’s greatest natural wonders.
Similar content being viewed by others
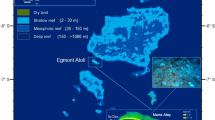
Mesophotic coral bleaching associated with changes in thermocline depth

Atypical weather patterns cause coral bleaching on the Great Barrier Reef, Australia during the 2021–2022 La Niña
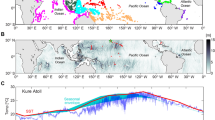
Internal tides can provide thermal refugia that will buffer some coral reefs from future global warming
Like many coral reefs globally, the World Heritage-listed GBR in Australia is under threat 4 , 6 . Mass coral bleaching, declining calcification rates 5 , 7 , outbreaks of crown-of-thorns starfish ( Acanthaster spp.) 8 , severe tropical cyclones 9 and overfishing 10 have placed compounding detrimental pressures on the reef ecosystem. Coral bleaching typically occurs when heat stress triggers the breakdown of the symbiosis between corals and their symbiotic dinoflagellates 11 . Although coral bleaching can occur locally as a result of low salinity, cold waters or pollution, regional and global mass bleaching events, in which the majority of corals in one or more regions bleach at once, are strongly associated with increasing SST linked to global warming 2 .
The first modern observations of mass coral bleaching on the GBR occurred in the 1980s, but these events were less widespread and generally less severe 3 than the bleaching events in the twenty-first century 4 . Stress bands in coral skeletal cores have provided potential evidence for pre-1980s bleaching in the GBR and Coral Sea, such as during the 1877–78 El Niño 12 . However, stress bands are evident in relatively few cores before 1980 (ref. 12 ), suggesting that severe mass bleaching did not occur in the 1800s and most of the 1900s.
As the oceans have warmed, however, mass coral bleaching events have become increasingly lethal to corals 4 . Coral bleaching on the GBR 1 in 1998 coincided with a strong eastern-Pacific El Niño, and in 2002 with a weak El Niño. El Niño events can induce lower cloud cover and increased solar irradiance over the GBR 13 , increasing the risk of thermal stress and mass bleaching events 14 . In 2004, water temperatures were anomalously warm, and although bleaching occurred in the Coral Sea 15 , it was not widespread in the GBR, probably because there was reduced upwelling and an associated reduced influence of nutrients on symbiotic dinoflagellate expulsion 16 .
However, in the nine January–March periods from 2016 to 2024 (inclusive) there were five mass coral bleaching events on the GBR. Each was associated with high SSTs and affected large sections of the reef. GBR mass bleaching occurred in both 2016 and 2017, influenced by the presence of an El Niño event in 2016, and led to the death of at least 50% of shallow-water (depths of 5–10 m) reef-building corals 4 . Major bleaching events occurred again in quick succession in 2020 and 2022, with the accumulated heat stress for large sections of the GBR reaching levels conducive to widespread bleaching but lower levels of coral mortality 1 . The bleaching event in 2022 occurred, unusually, during a La Niña event, which is typically associated with cooler summer SSTs, higher than average rainfall and higher cloud cover on the GBR 1 . At the time of writing, researchers are assessing the impacts of the 2024 mass bleaching event.
The frequency of recent mass coral bleaching and mortality on the GBR is cause for concern. In 2021, the World Heritage Committee of the United Nations Educational, Scientific and Cultural Organization (UNESCO) drafted 17 a decision to inscribe the GBR on the List of World Heritage in Danger, stating that the reef is “facing ascertained danger”, citing recent mass coral bleaching events and insufficient progress by the State Party (Australia) in countering climate change, improving water quality and land management issues. The committee’s adopted decisions 18 have not included inscription of the ‘in danger’ status, but the draft inscription highlights the seriousness of the recent mass coral bleaching events. Authorities in Australia 5 have noted that climate change and coral bleaching have deteriorated the integrity of the outstanding universal value of the GBR, a defining feature of its World Heritage status.
Although rapidly rising SSTs are attributed to human activities with virtual certainty 19 , understanding the multi-century SST history of the GBR is critical to understanding the influence of SST on mass coral bleaching and mortality in recent decades. Putting aside a problematic attempt to do this 20 , which was discredited 21 , 22 , knowledge of the long-term context for GBR SSTs comes primarily from two multi-century reconstructions based on the geochemistry of coral cores collected from the inner shelf 23 and outer shelf 24 (Flinders Reef) in the central GBR. These reconstructions showed that SSTs in the early 2000s were not unusually high relative to levels in the past three centuries, with five-year mean SSTs (and salinities) estimated to be higher in the 1700s than in the 1900s. However, these records were limited by their relatively coarse five-year sampling resolution and their most recent data point being from the early 2000s. After these studies were published, SSTs in the GBR have continued to rise. Updated analysis of coral data from Flinders Reef provides valuable improved temporal resolution 25 , but interpretations of these records remain limited spatially.
Here, we investigate the recent high SST events in the GBR region in the context of the past four centuries. We combine a network of 22 coral Sr/Ca and δ 18 O palaeothermometer series (Supplementary Tables 1 and 2 ) located in and near to the Coral Sea region to infer spatial mean SST anomalies (SSTAs) for January–March, the months when maximum SST and thermal bleaching are most likely to occur in the Coral Sea 16 , 26 , each year from 1618 to 1995 ( Methods and Supplementary Information ). Anthropogenic climate change began and proceeded entirely within the multi-century lives of some of these massive coral colonies, offering a continuous multi-century record covering the industrial era. We use this 1618–1995 reconstruction and the available 1900–2024 instrumental data to contextualize the modern trend and rank four centuries of January–March SSTAs with greater precision than was previously possible. We then assess the degree of human influence on ocean temperatures in the region using climate model simulations run both with and without anthropogenic forcing.
The instrumental period (1900–present)
Mass coral bleaching on the GBR in 2016, 2017, 2020, 2022 and 2024 during January–March coincided with widespread warm SSTAs in the surrounding seas 1 , including the Coral Sea (Fig. 1a–e , using ERSSTv5 data 27 ). The Coral Sea and GBR have experienced a strong warming trend since 1900 (Fig. 1f ). January–March SSTAs averaged over the GBR are strongly correlated ( ρ = 0.84, P ≪ 0.01) with those in the broader Coral Sea (Fig. 1f ), including when the long-term warming trend is removed from both time series ( ρ = 0.69, P < 0.01; Supplementary Fig. 4 ). Based on the strength of this correlation, we associate high January–March area-averaged Coral Sea SSTAs with increased thermal bleaching risk in the GBR.

a – e , SSTAs (using ERSSTv5 data) for January–March in the Australasian region relative to the 1961–90 average for the five recent GBR mass coral bleaching years: 2016, 2017, 2020, 2022 and 2024. The black box shows the Coral Sea region (4° S–26° S, 142° E–174° E). f , Coral Sea and GBR mean SSTAs for 1900–2024 in January–March relative to the 1961–90 average. The black vertical lines indicate the five recent GBR mass coral bleaching years.
Record temperatures were set in 2016 and 2017 in the Coral Sea, and in 2020 they peaked fractionally below the record high of 2017. The January–March of 2022 was another warm event, the fifth warmest on record at the time. Recent data (ERSSTv5) indicate that 2024 set a new record by a margin of more than 0.19 °C above the previous record for the region. The January–March mean SSTs averaged over the five mass bleaching years during the period 2016–2024 are 0.77 °C higher than the 1961–90 January–March averages in both the Coral Sea and the GBR. The multidecadal warming trend, extreme years and association between GBR and Coral Sea SSTs are similar for the HadISST 28 gridded SST dataset, with some notable differences in the 1900–40 period (Supplementary Fig. 3 ). Furthermore, analysis of modern temperature-sensitive Sr/Ca series from GBR corals for 1900–2017 provides coherent independent evidence of statistically significant multi-decadal warming trends in January–March SSTs in the central and southern GBR (Supplementary Information section 4.2 ).
A multi-century context (1618–present)
Reconstructing Coral Sea January–March SSTs from 1618 to 1995 extends the century-long instrumental record back in time by an additional three centuries (Fig. 2a and Methods ). The reconstruction (calibrated to ERSSTv5) shows that multi-decadal SST variability was a persistent feature in the past. At the centennial timescale, there is relative stability before 1900, with the exception that cooler temperatures prevailed in the 1600s. Warming during the industrial era has been evident since the early 1900s (Fig. 2a ). There is a warming trend for January–March of 0.09 °C per decade for 1900–2024 and 0.12 °C per decade for 1960–2024 (Fig. 1f ) using ERSSTv5 data. Calibrating our reconstruction to HadISST1.1 yields similar results, with some differences in the degree of pre-1900 variability at both multi-decadal and centennial timescales (Supplementary Information section 5.2.6 ).
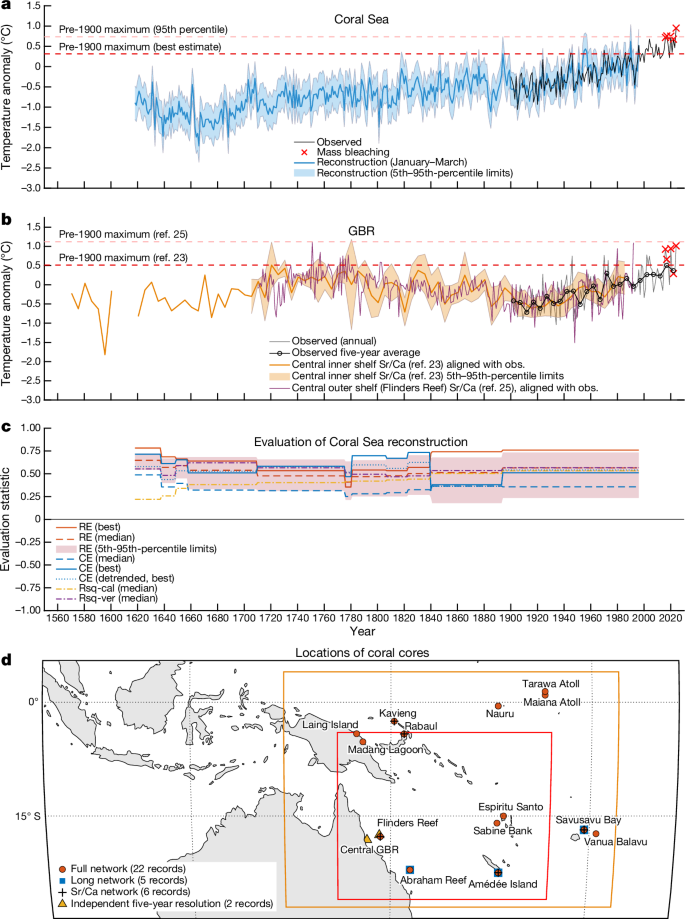
a , Reconstructed and observed mean January–March SSTAs in the Coral Sea for 1618–2024 relative to 1961–90. Dark blue, highest skill (maximum coefficient of efficiency) reconstruction with the full proxy network; light blue, 5th–95th-percentile reconstruction uncertainty; black, observed (ERSSTv5) data. Red crosses indicate the five recent mass bleaching events. Dashed lines indicate the best estimate (highest skill, red) and 95th-percentile (pink) uncertainty bound for the maximum pre-1900 January–March SSTA. b , Central GBR SSTA for the inner shelf 23 in thick orange and outer shelf 25 (Flinders Reef) in thin orange lines; these series are aligned here (see Methods ) with modern observations of mean GBR SSTAs for January–March relative to 1961–90. Observed data are shown at annual (grey line) and five-year (black line with open circles, plotted at the centre of each five-year period and temporally aligned with the five-year coral series 23 ) resolution. Dashed lines indicate best-estimate pre-1900 January–March maxima for refs. 23 (red) and 25 (pink). Orange shading indicates 5th–95th-percentile uncertainty bounds. Red crosses indicate the five recent mass bleaching events. c , Evaluation metrics for the Coral Sea reconstruction (Supplementary Information section 3.1 ); RE, reduction of error; CE, coefficient of efficiency; Rsq-cal, R-squared in the calibration period; Rsq-ver, R-squared in the verification (evaluation) period. d , Coral data locations relative to source data region (orange box) and Coral Sea region (red box). Coral proxy metadata are given in Supplementary Tables 1 and 2 .
Our best-estimate (highest skill; Methods ) annual-resolution Coral Sea reconstruction (Fig. 2a ), using the full coral network calibrated to the ERSSTv5 instrumental data, indicates that the January–March mean SSTAs in 2016, 2017, 2020, 2022 and 2024 were, respectively, 1.50 °C, 1.54 °C, 1.53 °C, 1.46 °C and 1.73 °C above the 1618–1899 (hereafter ‘pre-1900’) reconstructed average. Using the same best-estimate reconstruction, Coral Sea January–March SSTs during these GBR mass bleaching years were five of the six warmest years the region has experienced in the past 400 years (Fig. 2a ).
By comparing the recent warm events to the reconstruction’s uncertainty range ( Methods ), we quantify, using likelihood terminology consistent with recent reports from the Intergovernmental Panel on Climate Change 19 , that the recent heat extremes in 2017, 2020 and 2024 are ‘extremely likely’ (>95th percentile; Fig. 2a ) to be higher than any January–March in the period 1618–1899. Furthermore, the heat extremes in 2016 and 2022 are (at least) ‘very likely’ (>90th percentile) to be above the pre-1900 maximum. We perform a series of tests that verify that our findings are not simply an artefact of the nature of the coral network itself (Supplementary Information section 5.2 ). In a network perturbation test, we generate 22 subsets of the reconstruction by adding proxy records incrementally in order from the highest to the lowest correlation with the target (Supplementary Information section 5.2.5 ). We confirm that 2017, 2020 and 2024 were ‘extremely likely’ (>95th percentile) to have been warmer than any year pre-1900 (using ERSSTv5 data) for all of these proxy subsets. Furthermore, in 20 of the 22 subsets, 2016 was also ‘extremely likely’ (>95th percentile), rather than ‘very likely’, to be warmer (2022 was ‘extremely likely’ in 14 of the 22 subsets). All our additional tests, including a reconstruction with only Sr/Ca coral data (thereby omitting the possibility of any non-temperature signal in δ 18 O coral on the reconstruction), achieve high reconstruction skill and confirm the extraordinary nature of recent extreme temperatures in the multi-century context (Supplementary Information section 5.2 ). Analyses using HadISST1.1 generally show lower correlations with the coral data and reconstructions with slightly warmer regional SSTs before 1900, along with more-muted centennial and multi-decadal variability in the pre-instrumental period. Nevertheless, the HadISST1.1-calibrated reconstructions show that the recent thermal extremes are well above the best estimate (highest skill) of the pre-1900 maximum of reconstructed January–March SSTAs (Supplementary Fig. 42 ). Furthermore, lower SSTAs (in the HadISST1.1 data) relative to the previous three centuries (as in our reconstructions calibrated to HadISST1.1), coupled with the recently observed mass coral bleaching events, could indicate that long-lived corals have a greater sensitivity to warming than is currently recognized.
Reconstructed regional GBR SSTAs based on a five-year-resolution, multi-century coral δ 18 O record from the central inshore GBR 23 (Fig. 2b ) show similarly strong warming since 1900 but more multi-decadal-to-centennial variability than the Coral Sea reconstruction. Recent five-year mean January–March GBR SSTAs narrowly exceed the best estimate of the maximum pre-1900 five-year mean since the early 1600s (Fig. 2b ). The averages for the five-year periods centred on 2018 and 2022 exceed the pre-1900 maximum by 0.11 °C and 0.06 °C, respectively. Results are similar using the five-year-resolution Flinders Reef (central outer shelf) 24 record (Supplementary Fig. 24 ), although its interpretation is limited by the lack of uncertainty estimates available for that record. Our Coral Sea reconstruction incorporates an updated (annual resolution) record from Flinders Reef 25 , which indicates similar centennial trends (thin orange line in Fig. 2b ) and shows that the recent high January–March SSTA events have approached the estimated local pre-1900 maximum SSTA. Although contiguous multi-century cores from within the GBR are limited in their spatial extent, twentieth-century warming is evident in these records.
The extraordinary nature of the recent Coral Sea January–March SSTs in the context of the past 400 years is further illustrated by comparing the ranked temperature anomalies (Fig. 3 ) for the combined reconstructed and instrumental period from 1618–2024, incorporating reconstruction uncertainty ( Methods ). The mass coral bleaching years of 2016, 2017, 2020, 2022 and 2024, and the heat event of 2004, stand out as the warmest events across the whole 407-year record. The warmest three years (2024, 2017 and 2020) exceed the upper uncertainty bound (95th percentile) of the warmest reconstructed January–March in the pre-1900 period (pink (upper) dashed line in Fig. 3 ); 2016, 2004 and 2022 exceed the 90th percentile bound (red (lower) dashed line in Fig. 3 ). The warming trend is clear in the association between the ascending rank of the temperature anomalies and the year (shown as the colour of the filled circles in Fig. 3 ). Despite high interannual variability, 78 of the warmest 100 January–March periods between 1618 and 2024 occurred after 1900, and the 23 warmest all occur after 1900. The warmest 20 January–March periods all occur after 1950, coinciding with accelerated global warming.
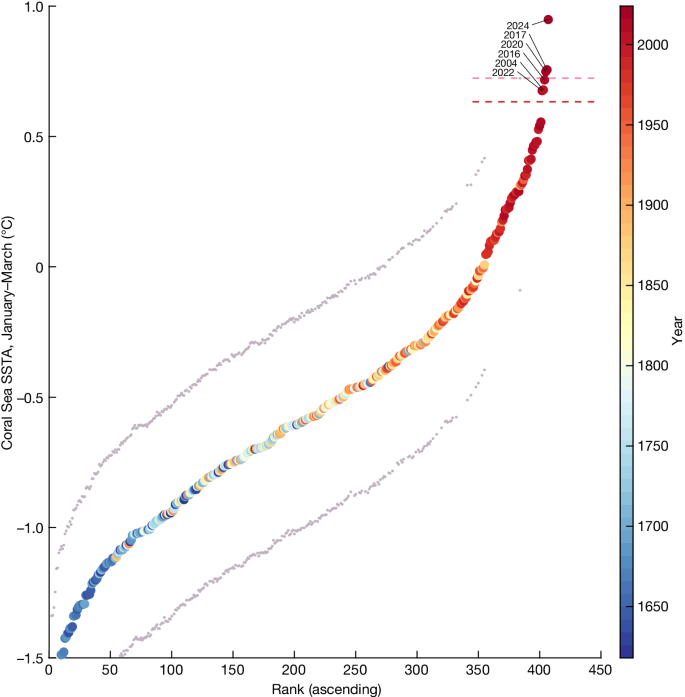
Ranked January–March SSTAs for 1618–2024 relative to 1961–90 (coloured circles) from the best-estimate (highest skill, full coral network) reconstruction (1618–1899) and instrumental (ERSSTv5) data (1900–2024). The year is indicated by the colour of the filled circles. The 5th–95th-percentile uncertainty bounds of the pre-1900 reconstructed SSTAs are shown by small grey dots. The year labels indicate the warmest six years on record, five of which were mass coral bleaching years on the GBR. The pink (upper) dashed line indicates the 95th-percentile uncertainty bound of the maximum pre-1900 reconstructed SSTA; the red (lower) dashed line indicates the 90th-percentile limit.
Assessing anthropogenic influence
Using climate model simulations from the most recent (sixth) phase of the Coupled Model Intercomparison Project 29 (CMIP6), we assess the human influence on January–March SSTAs in the Coral Sea. The model simulations are from two experiments in the Detection and Attribution Model Intercomparison Project (DAMIP) 30 . The first set of simulations represents historical climate conditions, including both the natural and human influences on the climate system over the 1850–2014 period (‘historical’; red in Fig. 4 ). The second experiment is a counterfactual climate that spans the same period and uses the same models but includes only natural influences on the climate, omitting all human influences (‘historical-natural’; blue in Fig. 4 ). The historical experiment includes anthropogenic emissions of greenhouse gases and aerosols, stratospheric ozone changes and anthropogenic land-use changes; the historical-natural experiment does not. Variations in natural climate forcings, such as from volcanic eruptions and solar variability, are incorporated in both experiments. We include models that have a transient climate response (the global mean surface-temperature anomaly at the time of a doubling of atmospheric CO 2 concentration) in the range 1.4–2.2 °C, which is deemed ‘likely’ by the science community 31 ( Methods and Supplementary Information ).

Climate-model simulations of Coral Sea January–March SSTAs relative to the 1850–1900 average for the period 1850–2014, for models within the ‘likely’ range for their transient climate response 31 . The blue line (median) and light blue shading (5th–95th-percentile limits) are from the ‘historical-natural’ climate model simulations (no anthropogenic climate forcing); the red line and light red shading are from the ‘historical’ simulations (anthropogenic influences on the climate included) using the same set of climate models. The climate-model-derived time of emergence of anthropogenic climate change, shown by the grey and black vertical lines (1976 and 1997), is when the ratio of the climate change signal to the standard deviation of noise/variability 32 across model ensemble members first rises above 1 and 2, respectively. All models are represented equally in the model ensemble.
It is only with the incorporation of anthropogenic influences on the climate that the model simulations capture the modern-era warming of the Coral Sea January–March SSTA (Fig. 4 ). The median of the historical simulations has statistically significant warming trends of 0.05 °C, 0.10 °C and 0.15 °C per decade for the periods from 1900, 1950 and 1970 to 2014, respectively; the equivalent historical-natural trends are smaller in magnitude than ±0.01 °C per decade. To further explore the centennial-scale trends, we use a bootstrap ensemble ( Methods ) of the two sets of 165-year simulations from 1850–2014. We found that 100% of the historical bootstrap ensemble has statistically significant positive trends ( Methods ) for 1900–2014, but this value is 0% for the historical-natural ensemble. The observed (ERSSTv5) mean SSTA for 2016–2024 of 0.60 °C relative to 1961–90 is warmer than any nine-year sequence in the 7,095 simulated years in the historical-natural experiments from models with transient climate responses in the ‘likely’ range 31 .
We also use the simulations to estimate the time of emergence of the anthropogenic influence on January–March Coral Sea SSTAs above the natural background variability. The anthropogenic warming signal 32 increases from near zero in 1900 to around 0.5 standard deviations of the variability (‘noise’) in 1960. The climate change signal-to-noise ratio then increases rapidly from 1960 to 2014, exceeding 1.0 in 1976, 2.0 in 1997 and around 2.8 by 2014, the end of these simulations (Fig. 4 , Methods and Supplementary Fig. 50 ). Anthropogenic impacts on the climate are virtually certain to be the primary driver of this long-term warming in the Coral Sea.
Previously, our knowledge of the SST history of the GBR and the Coral Sea region has been highly dependent on instrumental observations, with the exception of the five-year-resolution multi-century coral Sr/Ca and U/Ca SST reconstructions from the two point locations in the central GBR 23 , 24 , an update at one of these locations 25 , seasonal resolution ‘floating’ (in time) chronologies from the GBR in the Holocene 33 , 34 and point SST estimates further back in time 35 . Thus, the context of recent warming trends in the Coral Sea and GBR and their relation to natural variability on decadal to centennial timescales is largely unknown without reconstructions such as the one we developed here.
Our coral proxy network is located mostly beyond the GBR, in the Coral Sea, and some series are located outside the Coral Sea region (Fig. 2d ). The selection of the Coral Sea as a study region allowed for a larger sample of contributing coral proxy data than exists for the GBR. However, coral bleaching on the GBR can be influenced by factors other than large-scale SST, including local oceanic and atmospheric dynamics that can modulate the occurrence and severity of thermal bleaching and mortality events 13 . Nonetheless, warming of seasonal SSTs over the larger Coral Sea region is likely to prime the background state and increase the likelihood of smaller spatio-temporal-scale heat anomalies. Furthermore, where we use only the five-year resolution series directly from the GBR to reconstruct GBR SSTAs, we draw similar conclusions about the long-term trajectory of SSTAs as for our full coral network (Fig. 2b and Supplementary Fig. 24 ). Furthermore, short modern coral series from within the GBR, analysed in this study, document a multi-decadal warming signal that is coherent with instrumental data (Supplementary Figs. 29 and 30 ). Nonetheless, additional high-resolution, multi-century, temperature-sensitive coral geochemical series from within the GBR would help unravel the local and remote ocean–atmosphere contributions to past bleaching events and reduce uncertainties.
The focus on the larger Coral Sea study region also takes advantage of the global modelling efforts of CMIP6. The large number of ensemble members available for CMIP6 means that greater climate model diversity, and therefore greater certainty in our attribution analysis, is possible compared with most single model analyses. There is also a methodological benefit in having high replication of the same experiments run with multiple climate models. However, coarse-resolution global-scale models do not accurately simulate smaller-scale processes, such as inshore currents and mesoscale eddies in the Coral Sea or the Gulf of Carpentaria, which probably affect local surface temperatures and variations in nutrient upwelling in the GBR 36 , 37 . Upwelling on the GBR is linked to the strength of the East Australian Current 16 , the southward branch of the South Pacific subtropical gyre. The CMIP-scale models we use do capture these gyre dynamics. The models show that the East Australian Current is expected to increase in strength as the climate continues to warm through this century 38 , and this may lead to more nutrient inputs that can exacerbate coral sensitivity to rising heat stress 39 , 40 . As well as focusing our model analysis on the larger Coral Sea region, we use a three-month time step. In doing so, we minimize the impact of model spatio-temporal resolution on our inferences about the role of anthropogenic greenhouse-gas emissions on the SST conditions that give rise to GBR mass bleaching.
Remaining uncertainties
We present analyses and interpretations that are as robust as possible given currently available data and methods. However, several sources of remaining uncertainty mean that future reconstructions of past Coral Sea and GBR SSTs could differ from those presented here. Although bias corrections are applied to observational SST datasets such as ERSST and HadISST, these datasets probably retain biases, especially for the period during and before 1945 (ref. 41 ), and these may not be fully accounted for in the uncertainty estimates 42 . Because our reconstructions are calibrated directly to these datasets, future observational-bias corrections are likely to improve proxy-based reconstructions.
Reconstructions of SST that use coral δ 18 O records may be susceptible to the influence of changes in the coral δ 18 O–SST relationship on time periods longer than the instrumental training period, along with non-SST changes in the δ 18 O of seawater, which can covary with salinity. As such, new coral records of temperature-sensitive trace-element ratios such as Sr/Ca, Li/Mg or U/Ca may prove influential in future efforts to distinguish between changes in past temperature and hydroclimate. Owing to the limited availability of multi-century coral data from within the GBR itself, the reconstructed low-frequency variability of GBR SSTs in recent centuries is likely to change as more temperature proxy data become available. It is also likely that new sub-annual resolution records would aid in removing potential signal damping or bias from our use of some annual-resolution records to reconstruct seasonal SSTAs.
Ecological consequences
With global warming of 0.8–1.1 °C above pre-industrial levels 19 there has been a marked increase in mass coral bleaching globally 43 . Even limiting global warming to the Paris Agreement’s ambitious 1.5 °C level would be likely to lead to the loss of 70–90% of corals that are on reefs today 44 . If all current international mitigation commitments are implemented, global mean surface temperature is still estimated to increase in the coming decades, with estimates varying between 1.9 °C (ref. 45 ) and 3.2 °C (ref. 46 ) above pre-industrial levels by the end of this century. Global warming above 2 °C would have disastrous consequences for coral ecosystems 19 , 44 and the hundreds of millions of people who currently depend on them.
Coral reefs of the future, if they can persist, are likely to have a different community structure to those in the recent past, probably one with much less diversity in coral species 4 . This is because mass bleaching events have a differential impact on different coral species. For example, fast-growing branching and tabulate corals are affected more than slower-growing massive species because they have different thermal tolerance 4 . The simplification of reef structures will have adverse impacts on the many thousands of species that rely on the complex three-dimensional structure of reefs 4 . Therefore, even with an ambitious long-term international mitigation goal, the ecological function 4 of the GBR is likely to deteriorate further 5 before it stabilizes.
Coral adaptation and acclimatization may be the only realistic prospect for the conservation of some parts of the GBR this century. However, although adaptation opportunities may be plausible to some extent 47 , they are no panacea because evolutionary changes to fundamental variables such as temperature take decades, if not centuries, to occur, especially in long-lived species such as reef-building corals 48 . There is currently no clear evidence of the real-time evolution of thermally tolerant corals 48 . Most rapid changes depend on a history of exposure to key genetic types and extremes, and there are limitations to genetic adaptation that prevent species-level adaptation to environments outside of their ecological and evolutionary history 19 . Model projections also indicate that rates of coral adaptation are too slow to keep pace with global warming 49 . In a rapidly warming world, the temperature conditions that give rise to mass coral bleaching events are likely to soon become commonplace. So, although we may see some resilience of coral to future marine heat events through acclimatization, thermal refugia are likely to be overwhelmed 50 . Global warming of more than 1.5 °C above pre-industrial levels will probably be catastrophic for coral reefs 44 .
Our new multi-century reconstruction illustrates the exceptional nature of ocean surface warming in the Coral Sea today and the resulting existential risk for the reef-building corals that are the backbone of the GBR. The reconstruction shows that SSTs were relatively cool and stable for hundreds of years, and that recent January–March ocean surface heat in the Coral Sea is unprecedented in at least the past 400 years. The coral colonies and reefs that have lived through the past several centuries, and that yielded the valuable Sr/Ca and δ 18 O data on which our reconstruction is based, are themselves under serious threat. Our analysis of climate-model simulations confirms that human influence is the driver of recent January–March Coral Sea surface warming. Together, the evidence presented in our study indicates that the GBR is in danger. Given this, it is conceivable that UNESCO may in the future reconsider its determination that the iconic GBR is not in danger. In the absence of rapid, coordinated and ambitious global action to combat climate change, we will likely be witness to the demise of one of Earth’s great natural wonders.
Instrumental observations
The Coral Sea and GBR area-averaged monthly SSTAs relative to 1961–90 for January–March are obtained from version 5 of the Extended Reconstructed Sea Surface Temperature dataset (ERSSTv5) 27 . We compare our results using ERSSTv5 with those generated using the Hadley Centre Sea Ice and Sea Surface Temperature dataset (HadISST1.1) 28 . We use only post-1900 instrumental SST observations here. Although gridded datasets have some coverage before 1900, ship-derived temperature data in the region for that period are too sparse to be reliable for calibrating our reconstruction (Supplementary Information section 1.2 ). The regional mean for the GBR is computed using the seven grid-cell locations used by the Australian Bureau of Meteorology (Supplementary Information section 1.1 ). We define the Coral Sea region as the ocean areas inside 4° S–26° S, 142° E–174° E.
Coral-derived temperature proxy data
We use a network of 22 published and publicly available sub-annual and annual resolution temperature-sensitive coral geochemical series (proxies; Fig. 2d , Supplementary Tables 1 and 2 , and Supplementary Fig. 5a–v ) from the western tropical Pacific in our source data region (4° N–27° S, 134° E–184° E) that cover at least the period from 1900 to 1995. Of these 22 series, 16 are δ 18 O, which are in per mil (‰) notation relative to Vienna PeeDee Belemnite (VPDB) 51 ; the remaining six are Sr/Ca series. The coral data are used as predictors in the reconstruction of January–March mean SSTAs in the Coral Sea region. We apply the inverse Rosenblatt transformation 52 , 53 to the coral data to ensure that our reconstruction predictors are normally distributed. Sub-annually resolved series are converted to the annual time step by averaging across the November–April window. This maximizes the detection of the summer peak values, allowing for some inaccuracy in sub-annual dating and the timing of coral skeleton deposition 54 , 55 . A small fraction (less than 0.8%) of missing data is infilled using the regularized expectation maximization (RegEM) algorithm 56 (Supplementary Information section 2.3 ), after which the proxy series are standardized such that each has a mean of zero and a standard deviation of one over their common 1900–1995 period.
Reconstruction method
To produce our Coral Sea reconstruction, we use nested principal component regression 57 (PCR), in which the principal components of the network of 22 coral proxies are used as regressors against the target-region January–March SSTA relative to the 1961–90 average. We perform the reconstructions separately for each nest of proxies, where a nest is a set of proxies that cover the same time period. The longest nest dates back to 1618, when at least two series are available. The nests allow for the use of all coral proxies over the full time period of their coverage. The 96-year portion of the instrumental period (1900–1995) that overlaps with the reconstruction period is used for calibration and evaluation (or equivalently, verification) against observations. We reconstruct regional SSTAs from the principal components of the coral network of δ 18 O and Sr/Ca data, rather than their local SST calibrations, to minimize the number of computational steps and to aid in representing the full reconstruction uncertainty.
Principal component analysis (PCA) is used to reduce the dimensionality of the proxy matrix, as follows. Let P ( t , r ) denote the palaeoclimate-data matrix during the time period t = 1,..., n at an annual time step for proxy series r = 1,..., p . PCA is undertaken on this matrix during the calibration period, P cal . We obtain the principal component coefficients matrix P coeff ( r , e ) for principal components e = 1,..., n PC and principal component scores P score ( t , e ), which are representations of the input matrix P cal in the principal component space. P score is truncated to include n PC,use principal components to form \({P}_{{\rm{score}}}^{{\prime} }\) such that the variance of the proxy network explained by the n PC,use principal components is greater than \({\sigma }_{{\rm{expl}}}^{2}\) (which we set to 95%). Reconstruction tests in which \({\sigma }_{{\rm{expl}}}^{2}\) is varied from 70% to 95% show that our results are not strongly sensitive to this choice, and tests based on lag-one autoregressive noise for \({\sigma }_{{\rm{expl}}}^{2}\) from 50% to 99% further support this choice (Supplementary Information section 3.2 ). These principal components are used as predictors against which the Coral Sea January–March instrumental SSTAs are regressed. We regress the standardized SSTA target data during the calibration period, I cal , against the retained principal components of the predictor data, \({P}_{{\rm{score}}}^{{\prime} }\) :
Thus, we obtain n PC,use estimates of the regression coefficients γ e with gaussian error term ε t ~ N (0, \({\sigma }_{N}^{2}\) ). The principal components are extended back into the pre-instrumental period by multiplying the entire proxy matrix P ( t , p ) with the truncated principal component coefficient matrix \({P}_{{\rm{coeff}}}^{{\prime} }\) ( t , e ) to obtain \({Q}_{{\rm{coeff}}}^{{\prime} }\) :
The reconstruction proceeds with the fitted regression coefficients γ e and extended coefficient matrix \({Q}_{{\rm{coeff}}}^{{\prime} }\) to obtain a reconstruction time series R m ( t ) for a given nest of proxy series
The standardized reconstruction R m ( t ) is then calibrated to the instrumental data such that the standard deviation and mean of the reconstruction and target during the calibration interval are equal. As well as obtaining reconstructions for each nest of available proxies, we compute stitched reconstructions S c ( t ) for each calibration period c , which include at each time step the reconstructed data for the proxy nest with maximum coefficient of efficiency 58 , 59 (Supplementary Information section 3.1 ). This procedure is performed for contiguous calibration intervals between 60 and 80 years duration between 1900 and 1995, with interval width and location increments of two years, reserving the remaining data in the overlapping period for independent evaluation, and for all proxy nests. The reconstruction error is modelled with a lag-one autoregressive process fitted to the residuals. We evaluate the capacity of our reconstruction method to achieve spurious skill from overfitting by performing a test in which we replace the coral data with synthetic noise (Supplementary Information section 3.2i ). We find that reconstructions based on synthetic noise achieve extremely low or zero skill and as more noise principal components are included in the regression, the evaluation metrics indicate declining skill. Our reconstruction and evaluation methods therefore guard against the potential for spurious skill.
Pseudo-proxy reconstructions
Our reconstruction method is further evaluated by using a pseudo-proxy modelling approach based on the Community Earth System Model (CESM) Last Millennium Experiment (LME) 60 , for which there are 13 full-forcing ensemble members covering the period 850–2005. We use the pseudo-proxy reconstructions to evaluate our reconstruction method and coral network in a fully coupled climate-model environment. We form pseudo-proxies by extracting from each LME ensemble member the SST and sea surface salinity (SSS) from the 1.5° × 1.5° grid cell located nearest to our coral data. We then apply proxy system models in the form of linear regression models, basing δ 18 O on both SST and SSS, and Sr/Ca on SST only (Supplementary Information section 3.3 ). We set the spatial and temporal availability of the pseudo-coral network to match that of the coral network. We then apply our PCR reconstruction and evaluation procedure to the pseudo-proxy network, taking advantage of the availability of the modelled Coral Sea SSTA data across the multi-century period of 1618–2005, which allows for the evaluation of the pseudo-proxy reconstruction over this entire time period. We first test our method using a ‘perfect proxy’ approach (with no proxy measurement error) before superimposing synthetic noise on the pseudo-proxy time series, evaluating our methodology at two separate levels of measurement error, quantified by signal-to-noise ratios of 1.0 and 4.0. The evaluation metrics for these tests indicate that our coral network and reconstruction method obtain skilful reconstructions of Coral Sea SSTAs in the climate-model environment (Supplementary Figs. 17b , 18 , 20b , 21 , 22b and 23 ).
Comparison with independent coral datasets
We use two multi-century five-year-resolution coral series from the central GBR 23 , 24 (Fig. 2b and Supplementary Fig. 24 ) and a network of sub-annual and annual resolution modern coral series (dated from 1900 onwards but not covering the full 1900–1995 period) from 44 sites in the GBR (Supplementary Information section 4.2 ) for independent evaluation of coral-derived evidence for warming in the region. We estimate five-year GBR SSTAs (Fig. 2b ) by aligning the post-1900 mean and variance of the proxy and instrumental (ERSSTv5) data.
Reconstruction sensitivity to non-SST influences
Of the 22 available coral series, 16 are records of δ 18 O, a widely used measure of the ratio of the stable isotopes 18 O and 16 O. In the tropical Pacific Ocean, δ 18 O is significantly correlated with SST 61 , 62 , 63 , 64 . Coral δ 18 O is also sensitive to the δ 18 O of seawater 65 , which can reflect advection of different water masses and/or changes in freshwater input, such as from riverine sources or precipitation, which in turn co-vary with SSS. Thus, it is generally considered that the main non-SST contributions to coral δ 18 O are processes that co-vary with SSS 62 , 66 . Our methodology minimizes the influence of non-temperature impacts on the reconstruction by exploiting the contrast in spatial heterogeneity between SST and SSS in January–March (Supplementary Information section 5.1 ). SSS is spatially inhomogeneous in the tropical Pacific 66 , 67 , leading to low coherence in SSS signals across our coral network. By contrast, the strong and coherent SST signal across our coral network locations and the Coral Sea region leads to principal components that are strongly representative of SST variations. This produces a skilful reconstruction of SST, as determined by evaluation against independent observations, and low correlations with SSS across the Coral Sea region (Supplementary Fig. 31 ).
Although the likelihood of non-SST influences on our SST reconstruction is low, we nonetheless test the sensitivity of our reconstruction and its associated interpretations to the possibility of these influences on the coral data. The tests compute the correlations between our best-estimate SSTA reconstruction (highest coefficient of efficiency) and observations of SSS, along with a series of additional reconstructions based on subsets of our coral network. The correlations between our highest coefficient of efficiency January–March Coral Sea SSTA reconstruction and January–March SSS are mapped for the Coral Sea and its neighbouring domain using three instrumental SSS datasets (Supplementary Fig. 31 ). Correlations are not statistically significant over most of the domain. Noting differing spatial correlation patterns between the instrumental SSS datasets 68 , which also cover different time periods (Supplementary Information section 5.1 ), we undertake six sensitivity tests using subsets of the coral network (Supplementary Information section 5.2 ). We use the following combinations of coral series: (1) the full network of 22 δ 18 O and Sr/Ca series (Figs. 2a and 3 ); (2) a subset of the six available Sr/Ca series (Supplementary Figs. 32 – 33 ), to test how the reconstruction is influenced by the inclusion of coral δ 18 O records; (3) a fixed nest subset of the five longest coral series, extending back to at least 1700 (Supplementary Figs. 34 – 35 ), to test for the potential influence of combining series of differing lengths (from our splicing of portions of the best reconstructions from each nest); (4) a subset of the ten coral series that are most strongly correlated with the target (Supplementary Figs. 36 and 37 ), to test how our reconstruction is influenced by the inclusion of coral series that are less strongly correlated with our target; (5) a subset of coral series that excludes the six records that are reported to potentially include biological mediation or non-climatic effects, or have low correlation with the target (Supplementary Figs. 38 and 39 ), to test their influence on the reconstruction; and (6) a network perturbation test comprising 22 separate subsets of proxies, in which proxy records are added incrementally in order of highest to lowest correlation with the target, starting with a single coral series and increasing the number of included proxies to all 22 series in our network (Supplementary Information section 5.2.5 ), to systematically quantify the influence of gradually including more coral datasets on our reconstruction and its interpretations.
The evaluation metrics (Fig. 2c and Supplementary Figs. 32b , 34b , 36b and 38b ) indicate a skilful reconstruction back to 1618 for the reconstructions based on the Full, Sr/Ca only, Long, Best-10 and OmitBioMed networks. These reconstructions explain 82.7%, 80.6%, 77.6%, 79.8% and 80.4% (R-squared values) of the variance in January–March SSTAs, respectively, in the independent evaluation periods (using ERSSTv5b). All coral subsets in the network perturbation test produce skilful reconstructions (Supplementary Fig. 40 ). The highest-skill reconstructions for all subsets in the network perturbation test align with our key interpretations (Supplementary Figs. 41 and 42 ). Together, our sensitivity tests show that the coral network, observational data and reconstruction methodology are a sound basis for reconstructing Coral Sea January–March SSTAs in past centuries and contextualizing recent high-SST events ( Supplementary Information ).
Climate-model attribution ensembles and experiments
The multi-model attribution analysis used here is based on simulations from CMIP6. We analyse simulations from the historical experiment (including natural and anthropogenic influences for 1850–2014) and the historical-natural experiment (natural-only forcings for 1850–2014). We select climate models for which monthly surface temperature is available in at least three historical and historical-natural simulations (Supplementary Table 5 ). All model simulations are interpolated to a common regular 1.5° × 1.5° latitude–longitude grid. January–March SSTAs relative to 1961–90 are calculated for each simulation. The full historical all-forcings ensemble is composed of 14 models with 268 simulations for 1850–2014. The natural-only ensemble is composed of the same 14 models with 95 individual simulations. A subset of climate models in the CMIP6 ensemble are considered by the science community to be ‘too hot’, simulating warming in response to increased atmospheric carbon dioxide concentrations that is larger than that supported by independent evidence 31 . We omit these models from our analysis by including only models with a transient climate response in the ‘likely’ range 31 of 1.4–2.2 °C. Our results are not strongly sensitive to this selection (Supplementary Information section 6.3 ). The ten remaining models yield a total of 25,410 years from 154 historical ensemble members and 7,095 years from 43 historical-natural ensemble members. We weight the models equally in our analysis using bootstrap sampling. We report linear trends based on simple linear regression models fitted with ordinary least squares. The statistical significance of linear trends is assessed using the Spearman’s rank correlation test 69 .
Time of emergence of the anthropogenic impact
We assess the anthropogenic influence on SSTAs in the Coral Sea region by starting with the assumption that any anthropogenic influence on SSTAs in the Coral Sea is indistinguishable from natural variability at the commencement of the model experiments. We measure the impact of anthropogenic influence on the climate in the region using a signal-to-noise approach 32 , 70 . We calculate the anthropogenic ‘signal’ as the mean of the difference between the smoothed (using a 41-year Lowess filter) modelled historical Coral Sea SSTA and the mean smoothed modelled historical-natural SSTA. Our ‘noise’ is the standard deviation of the difference between the modelled historical SSTA and its smoothed time series (Supplementary Information section 6 ).
Methods additionally rely on Supplementary Information and refs. 71 , 72 , 73 , 74 , 75 , 76 , 77 , 78 , 79 , 80 , 81 , 82 , 83 , 84 , 85 , 86 , 87 , 88 , 89 , 90 , 91 , 92 , 93 , 94 , 95 , 96 , 97 , 98 , 99 , 100 , 101 , 102 , 103 , 104 .
Data availability
The ERSSTv5 instrumental SST data are available from the US National Oceanic and Atmospheric Administration at https://psl.noaa.gov/data/gridded/data.noaa.ersst.v5.html . The HadISST1.1 data are available from the UK Met Office at https://www.metoffice.gov.uk/hadobs/hadisst/ . The original coral palaeoclimate data are available at the links provided in Supplementary Table 2 . Land areas for maps are obtained from the Mapping Toolbox v.23.2 in Matlab v.2023b and the Global Self-consistent, Hierarchical, High-resolution Geography (GSHHS) Database at https://www.soest.hawaii.edu/pwessel/gshhg/ through the m_map toolbox by R. Pawlowicz, available at https://www.eoas.ubc.ca/%7Erich/map.html . Prepared data from the coral geochemical series, reconstructions and climate models that support the findings of this study are available at: https://doi.org/10.24433/CO.4883292.v1 .
Code availability
The code that supports the findings of this study is available and can be run at : https://doi.org/10.24433/CO.4883292.v1 .
Australian Institute of Marine Science. Long-Term Monitoring Program. https://www.aims.gov.au/research-topics/monitoring-and-discovery/monitoring-great-barrier-reef/long-term-monitoring-program (2024).
Hughes, T. P. et al. Global warming and recurrent mass bleaching of corals. Nature 543 , 373–377 (2017).
Article ADS CAS PubMed Google Scholar
Hoegh-Guldberg, O. Climate change, coral bleaching and the future of the world’s coral reefs. Mar. Freshw. Res. 50 , 839–866 (1999).
Google Scholar
Hughes, T. P. et al. Global warming transforms coral reef assemblages. Nature 556 , 492–496 (2018).
Great Barrier Reef Marine Park Authority. Great Barrier Reef Outlook Report 2019 (Great Barrier Reef Marine Park Authority, 2019).
Hughes, T. P. et al. Coral reefs in the Anthropocene. Nature 546 , 82–90 (2017).
Davis, K. L., Colefax, A. P., Tucker, J. P., Kelaher, B. P. & Santos, I. R. Global coral reef ecosystems exhibit declining calcification and increasing primary productivity. Commun. Earth Environ. 2 , 105 (2021).
Article ADS Google Scholar
Westcott, D. A. et al. Relative efficacy of three approaches to mitigate Crown-of-Thorns Starfish outbreaks on Australia’s Great Barrier Reef. Sci. Rep. 10 , 12594 (2020).
Article ADS CAS PubMed PubMed Central Google Scholar
Mellin, C. et al. Spatial resilience of the Great Barrier Reef under cumulative disturbance impacts. Glob. Chang. Biol. 25 , 2431–2445 (2019).
Article ADS PubMed Google Scholar
Jackson, J. B. C. et al. Historical overfishing and the recent collapse of coastal ecosystems. Science 293 , 629–637 (2001).
Article CAS PubMed Google Scholar
Hoegh-Guldberg, O. & Smith, G. J. The effect of sudden changes in temperature, light and salinity on the population density and export of zooxanthellae from the reef corals Stylophora pistillata Esper and Seriatopora hystrix Dana. J. Exp. Mar. Biol. Ecol. 129 , 279–303 (1989).
Article Google Scholar
DeCarlo, T. M. et al. Acclimatization of massive reef-building corals to consecutive heatwaves. Proc. Biol. Sci. 286 , 20190235 (2019).
PubMed PubMed Central Google Scholar
McGowan, H. & Theobald, A. ENSO weather and coral bleaching on the Great Barrier Reef, Australia. Geophys. Res. Lett. 44 , 10,601–10,607 (2017).
Zhao, W., Huang, Y., Siems, S. & Manton, M. The role of clouds in coral bleaching events over the Great Barrier Reef. Geophys. Res. Lett. 48 , e2021GL093936 (2021).
Oxley, W. G., Emslie, M., Muir, P. & Thompson, A. Marine Surveys Undertaken in the Lihou Reef National Nature Reserve (Australian Institute of Marine Science, 2004).
DeCarlo, T. M. & Harrison, H. B. An enigmatic decoupling between heat stress and coral bleaching on the Great Barrier Reef. PeerJ 7 , e7473 (2019).
Article PubMed PubMed Central Google Scholar
UNESCO World Heritage Committee. Extended 44th Session of the World Heritage Committee, Fuzhou (China) 16–31 July 2021 . Draft decision 44 COM 7B.90. https://whc.unesco.org/document/188005 (UNESCO, 2021).
UNESCO World Heritage Committee. Extended 45th Session of the World Heritage Committee, Riyadh (Saudi Arabia) 10–25 September 2023 . Decision 45 COM 7B.13. https://whc.unesco.org/document/199654 (UNESCO, 2023).
IPCC. Summary for Policymakers. In Climate Change 2021: The Physical Science Basis. Contribution of Working Group I to the Sixth Assessment Report of the Intergovernmental Panel on Climate Change (eds Masson-Delmotte, V. et al.) (Cambridge Univ. Press, 2021).
Kamenos, N. A. & Hennige, S. J. Reconstructing four centuries of temperature-induced coral bleaching on the Great Barrier Reef. Front. Mar. Sci. 5 , 283 (2018).
Hoegh-Guldberg, O. et al. Commentary: reconstructing four centuries of temperature-induced coral bleaching on the Great Barrier Reef. Front. Mar. Sci. 6 , 86 (2019).
DeCarlo, T. M. Commentary: reconstructing four centuries of temperature-induced coral bleaching on the Great Barrier Reef. Front. Mar. Sci. 7 , 30 (2020).
Hendy, E. J. et al. Abrupt decrease in tropical Pacific sea surface salinity at end of Little Ice Age. Science 295 , 1511–1514 (2002).
Calvo, E. et al. Interdecadal climate variability in the Coral Sea since 1708 A.D. Palaeogeogr. Palaeoclimatol. Palaeoecol. 248 , 190–201 (2007).
Zinke, J. et al. North Flinders Reef (Coral Sea, Australia) Porites sp. corals as a candidate global boundary stratotype section and point for the Anthropocene series. Anthropocene Rev. 10 , 201–224 (2023).
Spady, B. L. et al. Global Coral Bleaching Database (NCEI Accession 0228498) (NOAA National Centers for Environmental Information, 2022); https://www.ncei.noaa.gov/archive/accession/0228498 .
Huang, B. et al. Extended reconstructed sea surface temperature, Version 5 (ERSSTv5): Upgrades, validations, and intercomparisons. J. Clim. 30 , 8179–8205 (2017).
Rayner, N. A. et al. Global analyses of sea surface temperature, sea ice, and night marine air temperature since the late nineteenth century. J. Geophys. Res. Atmos. 108 , 4407 (2003).
Eyring, V. et al. Overview of the Coupled Model Intercomparison Project Phase 6 (CMIP6) experimental design and organization. Geosci. Model Dev. 9 , 1937–1958 (2016).
Gillett, N. P. et al. The Detection and Attribution Model Intercomparison Project (DAMIP v1.0) contribution to CMIP6. Geosci. Model Dev. 9 , 3685–3697 (2016).
Hausfather, Z., Marvel, K., Schmidt, G. A., Nielsen-Gammon, J. W. & Zelinka, M. Climate simulations: recognize the ‘hot model’ problem. Nature 605 , 26–29 (2022).
Hawkins, E. et al. Observed emergence of the climate change signal: from the familiar to the unknown. Geophys. Res. Lett. 47 , e2019GL086259 (2020).
Gagan, M. K. et al. Temperature and surface-ocean water balance of the mid-Holocene tropical western Pacific. Science 279 , 1014–1018 (1998).
Arzey, A. K. et al. Coral skeletal proxy records database for the Great Barrier Reef, Australia. Preprint at Earth Syst. Sci. Data https://doi.org/10.5194/essd-2024-159 (2024).
Brenner, L. D. et al. Coral record of Younger Dryas Chronozone warmth on the Great Barrier Reef. Paleoceanogr. Paleoclimatol. 35 , e2020PA003962 (2020).
Furnas, M. J. & Mitchell, A. W. Nutrient inputs into the central Great Barrier Reef (Australia) from subsurface intrusions of Coral Sea waters: a two-dimensional displacement model. Cont. Shelf Res. 16 , 1127–1148 (1996).
Wolanski, E., Andutta, F., Deleersnijder, E., Li, Y. & Thomas, C. J. The Gulf of Carpentaria heated Torres Strait and the Northern Great Barrier Reef during the 2016 mass coral bleaching event. Estuar. Coast. Shelf Sci. 194 , 172–181 (2017).
Oliver, E. C. J. & Holbrook, N. J. Extending our understanding of South Pacific gyre ‘spin-up’: modeling the East Australian Current in a future climate. J. Geophys. Res. Oceans 119 , 2788–2805 (2014).
DeCarlo, T. M. et al. Nutrient-supplying ocean currents modulate coral bleaching susceptibility. Sci. Adv. 6 , eabc5493 (2020).
Article ADS PubMed PubMed Central Google Scholar
Wiedenmann, J. et al. Nutrient enrichment can increase the susceptibility of reef corals to bleaching. Nat. Clim. Chang. 3 , 160–164 (2013).
Article ADS CAS Google Scholar
Chan, D. & Huybers, P. Correcting observational biases in sea surface temperature observations removes anomalous warmth during World War II. J. Clim. 34 , 4585–4602 (2021).
Chan, D., Kent, E. C., Berry, D. I. & Huybers, P. Correcting datasets leads to more homogeneous early-twentieth-century sea surface warming. Nature 571 , 393–397 (2019).
Hughes, T. P. et al. Spatial and temporal patterns of mass bleaching of corals in the Anthropocene. Science 359 , 80–83 (2018).
Hoegh-Guldberg, O. et al. Chapter 3: Impacts of 1.5°C global warming on natural and human systems. In Global Warming of 1.5°C (eds Masson-Delmotte, V. et al.) (IPCC, 2018).
Meinshausen, M. et al. Realization of Paris Agreement pledges may limit warming just below 2 °C. Nature 604 , 304–309 (2022).
Matthews, H. D. & Wynes, S. Current global efforts are insufficient to limit warming to 1.5 °C. Science 376 , 1404–1409 (2022).
Coles, S. L. et al. Evidence of acclimatization or adaptation in Hawaiian corals to higher ocean temperatures. PeerJ 6 , e5347 (2018).
Hughes, T. P., Baird, A. H., Morrison, T. H. & Torda, G. Principles for coral reef restoration in the anthropocene. One Earth 6 , 656–665 (2023).
Logan, C. A., Dunne, J. P., Ryan, J. S., Baskett, M. L. & Donner, S. D. Quantifying global potential for coral evolutionary response to climate change. Nat. Clim. Chang. 11 , 537–542 (2021).
Dixon, A. M., Forster, P. M., Heron, S. F., Stoner, A. M. K. & Beger, M. Future loss of local-scale thermal refugia in coral reef ecosystems. PLOS Clim. 1 , e0000004 (2022).
Coplen, T. B. Discontinuance of SMOW and PDB. Nature 375 , 285 (1995).
van Albada, S. J. & Robinson, P. A. Transformation of arbitrary distributions to the normal distribution with application to EEG test-retest reliability. J. Neurosci. Methods 161 , 205–211 (2007).
Article PubMed Google Scholar
Emile-Geay, J. & Tingley, M. Inferring climate variability from nonlinear proxies: application to palaeo-ENSO studies. Clim. Past 12 , 31–50 (2016).
Barnes, D. J., Taylor, R. B. & Lough, J. M. On the inclusion of trace materials into massive coral skeletons. Part II: distortions in skeletal records of annual climate cycles due to growth processes. J. Exp. Mar. Biol. Ecol. 194 , 251–275 (1995).
Article CAS Google Scholar
Gagan, M. K., Dunbar, G. B. & Suzuki, A. The effect of skeletal mass accumulation in Porites on coral Sr/Ca and δ 18 O paleothermometry. Paleoceanogr. Paleoclimatol. 27 , PA1203 (2012).
ADS Google Scholar
Schneider, T. Analysis of incomplete climate data: estimation of mean values and covariance matrices and imputation of missing values. J. Clim. 14 , 853–871 (2001).
PAGES 2k Consortium. Consistent multidecadal variability in global temperature reconstructions and simulations over the Common Era. Nat. Geosci. 12 , 643–649 (2019).
Cook, E. R., Briffa, K. R. & Jones, P. D. Spatial regression methods in dendroclimatology: a review and comparison of two techniques. Int. J. Climatol. 14 , 379–402 (1994).
Nash, J. E. & Sutcliffe, J. V. River flow forecasting through conceptual models part I − a discussion of principles. J. Hydrol. 10 , 282–290 (1970).
Otto-Bliesner, B. L. et al. Climate variability and change since 850 CE: an ensemble approach with the Community Earth System Model. Bull. Am. Meteorol. Soc. 97 , 735–754 (2016).
Evans, M. N., Kaplan, A. & Cane, M. A. Optimal sites for coral-based reconstruction of global sea surface temperature. Paleoceanogr. Paleoclimatol. 13 , 502–516 (1998).
Russon, T., Tudhope, A. W., Hegerl, G. C., Collins, M. & Tindall, J. Inter-annual tropical Pacific climate variability in an isotope-enabled CGCM: Implications for interpreting coral stable oxygen isotope records of ENSO. Clim. Past 9 , 1543–1557 (2013).
PAGES Hydro2k Consortium. Comparing proxy and model estimates of hydroclimate variability and change over the Common Era. Clim. Past 13 , 1851–1900 (2017).
Freund, M. B. et al. Higher frequency of Central Pacific El Niño events in recent decades relative to past centuries. Nat. Geosci. 12 , 450–455 (2019).
Gagan, M. K. et al. New views of tropical paleoclimates from corals. Quat. Sci. Rev. 19 , 45–64 (2000).
Thompson, D. M., Ault, T. R., Evans, M. N., Cole, J. E. & Emile-Geay, J. Comparison of observed and simulated tropical climate trends using a forward model of coral δ 18 O. Geophys. Res. Lett. 38 , L14706 (2011).
LeGrande, A. N. & Schmidt, G. A. Global gridded data set of the oxygen isotopic composition in seawater. Geophys. Res. Lett. 33 , L12604 (2006).
Reed, E. V., Thompson, D. M. & Anchukaitis, K. J. Coral-based sea surface salinity reconstructions and the role of observational uncertainties in inferred variability and trends. Paleoceanogr. Paleoclimatol. 37 , e2021PA004371 (2022).
Khaliq, M. N., Ouarda, T. B. M. J., Gachon, P., Sushama, L. & St-Hilaire, A. Identification of hydrological trends in the presence of serial and cross correlations: A review of selected methods and their application to annual flow regimes of Canadian rivers. J. Hydrol. 368 , 117–130 (2009).
Mahlstein, I., Hegerl, G. & Solomon, S. Emerging local warming signals in observational data. Geophys. Res. Lett. 39 , L21711 (2012).
Freeman, E. et al. ICOADS Release 3.0: a major update to the historical marine climate record. Int. J. Climatol. 37 , 2211–2232 (2017).
Huang, B. et al. Uncertainty estimates for sea surface temperature and land surface air temperature in NOAAGlobalTemp version 5. J. Clim. 33 , 1351–1379 (2020).
Druffel, E. R. M. & Griffin, S. Variability of surface ocean radiocarbon and stable isotopes in the southwestern Pacific. J. Geophys. Res. 104 , 23607–23613 (1999).
DeLong, K. L., Quinn, T. M., Taylor, F. W., Lin, K. & Shen, C.-C. Sea surface temperature variability in the southwest tropical Pacific since AD 1649. Nat. Clim. Change 2 , 799–804 (2012).
Quinn, T. et al. A multicentury stable isotope record from a New Caledonia coral: Interannual and decadal SST variability in the southwest Pacific since 1657. Paleoceanography 13 , 412–426 (1998).
Quinn, T. M., Crowley, T. J. & Taylor, F. W. New stable isotope results from a 173-year coral from Espiritu Santo, Vanuatu. Geophys. Res. Lett. 23 , 3413–3416 (1996).
Alibert, C. & Kinsley, L. A 170-year Sr/Ca and Ba/Ca coral record from the western Pacific warm pool: 1. What can we learn from an unusual coral record? J. Geophys. Res. Oceans 113 , C04008 (2008).
Tudhope, A. W. et al. Variability in the El Niño-Southern Oscillation through a glacial-interglacial cycle. Science 291 , 1511–1517 (2001).
Urban, F. E., Cole, J. E. & Overpeck, J. T. Influence of mean climate change on climate variability from a 155-year tropical Pacific coral record. Nature 407 , 989–993 (2000).
Guilderson, T. P. & Schrag, D. P. Reliability of coral isotope records from the western Pacific warm pool: A comparison using age-optimized records. Paleoceanography 14 , 457–464 (1999).
Quinn, T. M., Taylor, F. W. & Crowley, T. J. Coral-based climate variability in the Western Pacific Warm Pool since 1867. J. Geophys. Res. 111 , C11006 (2006).
Gorman, M. K. et al. A coral-based reconstruction of sea surface salinity at Sabine Bank, Vanuatu from 1842 to 2007 CE. Paleoceanography 27 , PA3226 (2012).
Bagnato, S., Linsley, B. K., Howe, S. S., Wellington, G. M. & Salinger, J. Coral oxygen isotope records of interdecadal climate variations in the South Pacific Convergence Zone region. Geochem. Geophys. Geosyst. 6 , Q06001 (2005).
Linsley, B. K. et al. Tracking the extent of the South Pacific Convergence Zone since the early 1600s. Geochem. Geophys. Geosyst. 7 , Q05003 (2006).
Cole, J. E., Fairbanks, R. G. & Shen, G. T. Recent variability in the Southern Oscillation: Isotopic results from a Tarawa Atoll coral. Science 260 , 1790–1793 (1993).
Dassié, E. P. et al. A Fiji multi-coral δ 18 O composite approach to obtaining a more accurate reconstruction of the last two-centuries of the ocean-climate variability in the South Pacific Convergence Zone region. Paleoceanography 29 , 1196–1213 (2014).
Carton, J. A., Chepurin, G. A. & Chen, L. SODA3: A new ocean climate reanalysis. J. Clim. 31 , 6967–6983 (2018).
Zuo, H., Balmaseda, M. A., Tietsche, S., Mogensen, K. & Mayer, M. The ECMWF operational ensemble reanalysis-analysis system for ocean and sea ice: A description of the system and assessment. Ocean Sci. 15 , 779–808 (2019).
Cheng, L. et al. Improved estimates of changes in upper ocean salinity and the hydrological cycle. J. Clim. 33 , 10357–10381 (2020).
Thompson, D. M. et al. Identifying hydro‐sensitive coral δ18O records for improved high‐resolution temperature and salinity reconstructions. Geophys. Res. Lett. 49 , e2021GL096153 (2022).
Wu, Y., Fallon, S. J., Cantin, N. E. & Lough, J. M. Assessing multiproxy approaches (Sr/Ca, U/Ca, Li/Mg, and B/Mg) to reconstruct sea surface temperature from coral skeletons throughout the Great Barrier Reef. Sci. Total Environ. 786 , 147393 (2021).
Sadler, J., Webb, G. E., Leonard, N. D., Nothdurft, L. D. & Clark, T. R. Reef core insights into mid-Holocene water temperatures of the southern Great Barrier Reef. Paleoceanography 31 , 1395–1408 (2016).
Roche, R. C. et al. Mid-Holocene sea surface conditions and riverine influence on the inshore Great Barrier Reef. Holocene 24 , 885–897 (2014).
Reed, E. V., Cole, J. E., Lough, J. M., Thompson, D. & Cantin, N. E. Linking climate variability and growth in coral skeletal records from the Great Barrier Reef. Coral Reefs 38 , 29–43 (2019).
Razak, T. B. et al. Use of skeletal Sr/Ca ratios to determine growth patterns in a branching coral Isopora palifera. Mar. Biol. 164 , 96 (2017).
Marshall, J. F. Decadal-scale, High Resolution Records of Sea Surface Temperature in the Eastern Indian and South Western Pacific Oceans from Proxy Records of the Strontium/calcium Ratio of Massive Porites Corals PhD thesis, Australian National Univ. (2000).
Marshall, J. F. & McCulloch, M. T. An assessment of the Sr/Ca ratio in shallow water hermatypic corals as a proxy for sea surface temperature. Geochim. Cosmochim. Acta 66 , 3263–3280 (2002).
Gagan, M. K. et al. Coral oxygen isotope evidence for recent groundwater fluxes to the Australian Great Barrier Reef. Geophys. Res. Lett. 29 , 43-1–43-4 (2002).
D’Olivo, J. P., Sinclair, D. J., Rankenburg, K. & McCulloch, M. T. A universal multi-trace element calibration for reconstructing sea surface temperatures from long-lived Porites corals: Removing ‘vital-effects’. Geochim. Cosmochim. Acta 239 , 109–135 (2018).
Fallon, S. J., McCulloch, M. T. & Alibert, C. Examining water temperature proxies in Porites corals from the Great Barrier Reef: a cross-shelf comparison. Coral Reefs 22 , 389–404 (2003).
Brenner, L. D., Linsley, B. K. & Potts, D. C. A modern Sr/Ca-δ 18 O-sea surface temperature calibration for Isopora corals on the Great Barrier Reef. Paleoceanography 32 , 182–194 (2017).
Alibert, C. et al. Source of trace element variability in Great Barrier Reef corals affected by the Burdekin flood plumes. Geochim. Cosmochim. Acta 67 , 231–246 (2003).
Murty, S. A. et al. Spatial and temporal robustness of Sr/Ca-SST calibrations in Red Sea corals: Evidence for influence of mean annual temperature on calibration slopes. Paleoceanogr. Paleoclimatol. 33 , 443–456 (2018).
Sayani, H. R., Cobb, K. M., DeLong, K., Hitt, N. T. & Druffel, E. R. M. Intercolony δ 18 O and Sr/Ca variability among Porites spp. corals at Palmyra Atoll: Toward more robust coral-based estimates of climate. Geochem. Geophys. Geosyst. 20 , 5270–5284 (2019).
Otto, F. E. L. Geert Jan van Oldenborgh 1961–2021. Nat. Clim. Chang. 11 , 1017 (2021).
Download references
Acknowledgements
We acknowledge the originators of the coral data cited in Supplementary Tables 1 and 2 ; S. E. Perkins-Kirkpatrick and the deceased G. J. van Oldenborgh 105 for contributions to an earlier version of this manuscript; E. P. Dassié and J. Zinke for discussions and data; R. Neukom for advice on an earlier version of the reconstruction code; and B. Trewin and K. Braganza for advice about the Bureau of Meteorology GBR SST time series. B.J.H. and H.V.M. acknowledge support from an Australian Research Council (ARC) SRIEAS grant, Securing Antarctica’s Environmental Future (SR200100005), and ARC Discovery Project DP200100206. A.D.K. acknowledges support from an ARC DECRA (DE180100638) and the Australian government’s National Environmental Science Program. B.J.H. and A.D.K. acknowledge an affiliation with the ARC Centre of Excellence for Climate Extremes (CE170100023). H.V.M. acknowledges support from an ARC Future Fellowship (FT140100286). A.K.A. acknowledges support from an Australian government research training program scholarship and an AINSE postgraduate research award. Funding was provided to B.K.L. by the Vetlesen Foundation through a gift to the Lamont-Doherty Earth Observatory. Grants to B.K.L. enabled the generation of coral oxygen isotope and Sr/Ca data from Fiji that were used in our reconstruction (US National Science Foundation OCE-0318296 and ATM-9901649 and US National Oceanic and Atmospheric Administration NA96GP0406). We acknowledge the support of the NCI facility in Australia and the World Climate Research Programme’s working group on coupled modelling, which is responsible for CMIP. We thank the climate-modelling groups for producing and making available their model output. For CMIP, the US Department of Energy’s Program for Climate Model Diagnosis and Intercomparison provided coordinating support and led the development of software infrastructure in partnership with the Global Organisation for Earth System Science Portals.
Author information
Authors and affiliations.
Environmental Futures, School of Earth, Atmospheric and Life Sciences, University of Wollongong, Wollongong, New South Wales, Australia
Benjamin J. Henley, Helen V. McGregor & Ariella K. Arzey
Securing Antarctica’s Environmental Future, University of Wollongong, Wollongong, New South Wales, Australia
School of Agriculture, Food and Ecosystem Sciences, University of Melbourne, Parkville, Victoria, Australia
Benjamin J. Henley
School of Geography, Earth and Atmospheric Sciences, University of Melbourne, Parkville, Victoria, Australia
Andrew D. King & David J. Karoly
ARC Centre of Excellence for Climate Extremes, University of Melbourne, Parkville, Victoria, Australia
Andrew D. King
School of the Environment, The University of Queensland, Brisbane, Queensland, Australia
Ove Hoegh-Guldberg
Australian Institute of Marine Science, Townsville, Queensland, Australia
Janice M. Lough
ARC Centre of Excellence for Coral Reef Studies and School of Earth Sciences, University of Western Australia, Crawley, Western Australia, Australia
Thomas M. DeCarlo
Department of Earth and Environmental Sciences, Tulane University, New Orleans, LA, USA
Lamont-Doherty Earth Observatory of Columbia University, Palisades, NY, USA
Braddock K. Linsley
You can also search for this author in PubMed Google Scholar
Contributions
B.J.H., H.V.M. and A.D.K. conceived the study and developed the methodology. B.J.H. did most of the analysis. A.K.A. contributed analysis of modern coral data (Supplementary Information section 4.2 ). T.M.D. contributed analysis of instrumental data coverage (Supplementary Information section 1.2 ). B.K.L. contributed sub-annual coral data. B.J.H. and H.V.M. led the preparation of the manuscript, with contributions from A.D.K., O.H.-G., A.K.A., D.J.K., J.M.L., T.M.D. and B.K.L. Generative artificial intelligence was not used in any aspect of this study or manuscript.
Corresponding author
Correspondence to Benjamin J. Henley .
Ethics declarations
Competing interests.
The authors declare no competing interests.
Peer review
Peer review information.
Nature thanks Simon Michel, Miriam Pfeiffer, Claudia Tebaldi and the other, anonymous, reviewer(s) for their contribution to the peer review of this work.
Additional information
Publisher’s note Springer Nature remains neutral with regard to jurisdictional claims in published maps and institutional affiliations.
Supplementary information
Supplementary information ., rights and permissions.
Open Access This article is licensed under a Creative Commons Attribution 4.0 International License, which permits use, sharing, adaptation, distribution and reproduction in any medium or format, as long as you give appropriate credit to the original author(s) and the source, provide a link to the Creative Commons licence, and indicate if changes were made. The images or other third party material in this article are included in the article’s Creative Commons licence, unless indicated otherwise in a credit line to the material. If material is not included in the article’s Creative Commons licence and your intended use is not permitted by statutory regulation or exceeds the permitted use, you will need to obtain permission directly from the copyright holder. To view a copy of this licence, visit http://creativecommons.org/licenses/by/4.0/ .
Reprints and permissions
About this article
Cite this article.
Henley, B.J., McGregor, H.V., King, A.D. et al. Highest ocean heat in four centuries places Great Barrier Reef in danger. Nature 632 , 320–326 (2024). https://doi.org/10.1038/s41586-024-07672-x
Download citation
Received : 02 November 2022
Accepted : 04 June 2024
Published : 07 August 2024
Issue Date : 08 August 2024
DOI : https://doi.org/10.1038/s41586-024-07672-x
Share this article
Anyone you share the following link with will be able to read this content:
Sorry, a shareable link is not currently available for this article.
Provided by the Springer Nature SharedIt content-sharing initiative
This article is cited by
Great barrier reefâs temperature soars to 400-year high.
- Jeff Tollefson
Nature (2024)
By submitting a comment you agree to abide by our Terms and Community Guidelines . If you find something abusive or that does not comply with our terms or guidelines please flag it as inappropriate.
Quick links
- Explore articles by subject
- Guide to authors
- Editorial policies
Sign up for the Nature Briefing: Anthropocene newsletter — what matters in anthropocene research, free to your inbox weekly.


IMAGES
COMMENTS
Abstract. Climate change is a long-lasting change in the weather arrays across tropics to polls. ... gathered the information in this report from various media outlets, research agencies, policy papers, newspapers, and other sources. ... Worldwide observed and anticipated climatic changes for the twenty-first century and global warming are ...
The paper introduces global warming, elaborates its causes and hazards and presents some solutions to solve this hot issue. Above all, alternative energy sources (solar, wind, hydro, geothermal ...
Abstract. Climate change and global warming have emerged as pressing challenges of our time, with far-reaching consequences for the environment, societies, and economies worldwide. This research ...
Abstract. Improved knowledge of glacial-to-interglacial global temperature change yields Charney (fast-feedback) equilibrium climate sensitivity 1.2 ± 0.3°C (2σ) per W/m 2, which is 4.8°C ± 1.2°C for doubled CO 2.Consistent analysis of temperature over the full Cenozoic era—including 'slow' feedbacks by ice sheets and trace gases—supports this sensitivity and implies that CO 2 ...
Background: Anthropogenic global warming, interacting with social and other environmental determinants, constitutes a profound health risk. This paper reports a comprehensive literature review for 1989-2013 (inclusive), the first 25 years in which this topic appeared in scientific journals. ... followed by the abstract of each paper, assessed ...
This wider set of search terms yielded a total of 88125 papers, whereas C13 identified a total of 11944 abstracts from papers published over the years 1991 and 2011. (Using our expanded search terms over the same 1991-2011 time period as C13 would have yielded 30627 results.) ... Implies humans are causing global warming. e.g. research ...
These scenarios yield a steep, relentless increase in global temperature throughout the twenty-first century ( 4, 10) with warming of several degrees Celsius by 2100, if climate sensitivity is 2-4°C for doubled CO 2, as climate models suggest ( 4, 11 - 13 ). These figures can give the impression that curtailment of global warming is almost ...
To achieve long-term climate change mitigation and adaptation goals, such as limiting global warming to 1.5 or 2 °C, there must be a global effort to decide and act upon effective but realistic ...
Prior to the mid-19th century, Earth was in the grip of the Little Ice Age. Since then, temperatures have on average trended upward. At the same time, human emissions of carbon dioxide (CO 2) have increased, and the interest of scientists has turned to consider the extent of the relative contributions of anthropogenic CO 2 and natural forces to warming.
The Earth may have left a safe climate state beyond 1°C global warming. A significant likelihood of passing multiple climate tipping points exists above ~1.5°C, particularly in major ice sheets. Tipping point likelihood increases further in the Paris range of 1.5 to <2°C warming. Current policies leading to ~2 to 3°C warming are unsafe ...
As the evidence for human-caused climate change has increased, the number of Americans who believe it has decreased. The latest Pew Research Center (2010) poll in October, 2009, shows that only 57% of Americans believe global warming is real, down from 71% in April, 2008.
Among abstracts expressing a position on AGW, 97.1% endorsed the consensus position that humans are causing global warming. In a second phase of this study, we invited authors to rate their own papers. Compared to abstract ratings, a smaller percentage of self-rated papers expressed no position on AGW (35.5%).
The Economic Geography of Global Warming José-Luis Cruz and Esteban Rossi-Hansberg NBER Working Paper No. 28466 February 2021 JEL No. F63,F64,Q51,Q54,Q56 ABSTRACT Global warming is a worldwide and protracted phenomenon with heterogeneous local economic effects. In order to evaluate the aggregate and local economic consequences of higher
levels increase by about 1-3% per degree Celsius of global warming1. This change is not spatially homogeneous: for instance, et regions are projected to have the largest future rise in rainfall2 ...
research articles. Research articles. Filter By: Article Type. All. All; Analysis (8) ... Matters Arising (2) Year. 2021 (115) All; 2021 (115) Global warming decreases connectivity among coral ...
That hypothesis was tested by analyzing 928 abstracts, published in refereed scientific journals between 1993 and 2003, and listed in the ISI database with the keywords "climate change" . The 928 papers were divided into six categories: explicit endorsement of the consensus position, evaluation of impacts, mitigation proposals, methods ...
To better understand the problem, gathered the information in this report from various media outlets, research agencies, policy papers, newspapers, and other sources. This review is a sectorial assessment of climate change mitigation and adaptation approaches worldwide in the aforementioned sectors and the associated economic costs.
Global temperature in the GISS analysis increased 0.28°C in 2023, from 1.16°C to 1.44°C (Fig. 1), the largest annual increase in the 144-year record. This annual rise is largely due to the ongoing tropical El Nino warming, but no prior El Nino engendered as much warming, which points to an additional drive for global warming acceleration.
Abstract: Energy and environment, particularly the global warming problem due to man-made greenhouse gases (GHGs), appear to be such a serious concern in our society that almost everybody in the world today is talking about it. The 2007 Nobel Peace Prize was awarded to the United Nations--Intergovernmental Panel of Climate Change (IPCC) along with the former U.S. Vice President Al Gore for ...
Background Climate change is safe to be one of the biggest challenges of mankind. Human activities, especially the combustion of fossil fuels, contribute to the increase of greenhouse gases in the atmosphere and thus to the pace of climate change. The effects of climate change are already being felt, and the resulting damage will most likely be enormous worldwide. Because global impacts vary ...
Additionally, the global warming trend continued to intensify after the end of global warming hiatus in 2013, but the changes of air surface freezing-thawing index (AFDD/ATDD) and permafrost extent in pan-Arctic in the decades preceding and following 2013 remain also unclear.
Abstract. Sea-level rise is a major effect of climate change. It has drawn international attention, because higher sea levels in the future would cause serious impacts in various parts of the world. There are questions associated with sea-level rise which science needs to answer. To what extent did climate change contribute to sea-level rise in ...
While animal-based (52%) and plant-based (48%) products contribute nearly equally to global dietary emissions 4,16, the latter accounts for 87% of calories in global diets (Supplementary Table 4 ...
The IO and AO SST anomalies in the global warming mode can affect on the changes in equatorial zonal circulations, but the influence of PO SST disturbs the changes in Indian Walker Circulation and ...
By 2023, the realized global warming due to IMO2020 changes is about +0.04 K, which is about 50% of the long term response to IMO2020 of about +0.07 K. This warming is 17% of the total anthropogenic warming from 2020 expected by 2023, and 13% of the total simulated anthropogenic global warming by 2030 (Figure 2b). Note that the forcing is ...
Carbon dioxide (CO 2), resulting from the combustion of fossil fuels, is widely recognized as one of the main contributors to global warming.However, the existing carbon capture methods lack stability and cost-effectiveness. In order to tackle this issue, lithium-carbon dioxide batteries (LCBs) have emerged as a promising solution due to their high energy density and environmentally friendly ...
To verify if the search string used for CC/GW/CE research may have missed a substantial portion of research papers that did not use the three searched keywords, a third search was conducted using the following search string: TOPIC: ((("greenhouse gas∗" OR "GHG∗") NOT ("climate" OR "climate change" OR "global warming" OR "climate emergency"))).
Even limiting global warming to the Paris Agreement's ambitious 1.5 °C level would be likely to lead to the loss of 70-90% of corals that are on reefs today 44.
Objective To quantify global intakes of sugar sweetened beverages (SSBs) and trends over time among children and adolescents. Design Population based study. Setting Global Dietary Database. Population Children and adolescents aged 3-19 years in 185 countries between 1990 and 2018, jointly stratified at subnational level by age, sex, parental education, and rural or urban residence. Results In ...